About Tissue Engineering Essay
Introduction, tissue engineering, works cited.
Tissue engineering is a discovery in the field of science. It was once a sub-field of biomaterials but due to considerable improvements in the sub-field, it is now a field on its own. Regenerative medicine is also used in place of this term. This is the process whereby cells and a combination of other factors are engineered. This is done to provide improvement or replacement of biological functions. It is the process whereby certain parts of the body tissues or an organ as a whole, are repaired or replaced. The tissues involved include the skin, bladder, bones, blood vessels, or cartilage (Langer and Vacanti 920).
Langer and Vacanti defined tissue engineering as “an interdisciplinary field that applies the principles of engineering and life science towards the development of biological substitutes that restore, maintain, or improve tissue functions or a whole organ” (922). It has also been defined as an “understanding of the principles of tissue growth, and its application to produce functional replacement tissue for clinical use” (Langer and Vacanti 923). The tissues that are usually involved in this process are those that require structural and mechanical properties to function properly. It can also be said to be the process of using cells to perform certain biochemical functions. This is done within a support system that has been created artificially. The artificially made organs include the liver (bio-artificial liver) and pancreas (artificial pancreas).
Through tissue engineering, various interventions have been made in the field of medicine. This has led to the production of tissue replacement parts. However, challenges have also faced this new intervention. One of them includes the need for more complex functionality. Another issue is in the functionality and stability of the artificially made tissues. Further research is needed to finally develop the human replacement tissues or organs that would match the actual organs.
There are several examples of tissues that have been derived from tissue engineering. One example is in vitro meat. This edible muscle tissue was developed artificially. It was cultured in vitro . An artificial pancreas was developed using islet cells. It was used to produce and regulate insulin in the body. This was important particularly to the persons who had diabetes. Artificially made bladders have also been tested and proven to be successful. Knee cartilages have also been repaired using tissues that had been engineered in the laboratories. Other examples include tissue-engineered airways, artificial skin, artificial bone marrow, artificial penises, and many more.
This form of innovation (tissue engineering) uses the body cells as the raw materials. Examples of the cells used include the chondrocytes and fibroblasts. These are used for repairing cartilages and the skin respectively. To extract the required cells, centrifugation is conducted on the fluid tissue. This includes the blood. When extracting from solid tissue, the tissue must first be minced. It is then digested using some enzymes to release the cells.
When the cells have been extracted, they are placed in a bioreactor where they can grow in vivo. A bioreactor provides an environment conducive for the process and the required tissues are produced.
Tissue engineering is the process of producing artificial tissues. It is necessary for repairing tissues or replacing organs in the human body. This innovation has brought success in medicine since it has helped in the engineering of necessary tissues such as the skin, bone marrow, bones, and pancreas. Several challenges face this concept but with more research, these challenges can be eliminated.
Langer, Robert, and Joseph Vacanti. “Tissue Engineering.” Science 260.5110 (1993): 920-926. Print.
- Private Practice of Nurse Practitioners in the USA
- Ethical Issues in Tissue Engineering
- How to Treat Wastewater
- Maintaining and Repairing Relationships
- Artificial Pancreas: Components, Challenges
- The Benefits of Sharing Knowledge About Diabetes With Physicians
- Meta-Analysis: The Critical Links of Success
- Qualitative Research and Evaluation Methods
- Ethics and Peer Review in Science
- The Power of Followership: Benefits, Individual Reflective Cycle
- Chicago (A-D)
- Chicago (N-B)
IvyPanda. (2022, April 29). About Tissue Engineering. https://ivypanda.com/essays/about-tissue-engineering/
"About Tissue Engineering." IvyPanda , 29 Apr. 2022, ivypanda.com/essays/about-tissue-engineering/.
IvyPanda . (2022) 'About Tissue Engineering'. 29 April.
IvyPanda . 2022. "About Tissue Engineering." April 29, 2022. https://ivypanda.com/essays/about-tissue-engineering/.
1. IvyPanda . "About Tissue Engineering." April 29, 2022. https://ivypanda.com/essays/about-tissue-engineering/.
Bibliography
IvyPanda . "About Tissue Engineering." April 29, 2022. https://ivypanda.com/essays/about-tissue-engineering/.
- To find inspiration for your paper and overcome writer’s block
- As a source of information (ensure proper referencing)
- As a template for you assignment
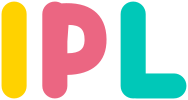

Wait a second!
More handpicked essays just for you.

About Tissue engineering
Tissue engineering pros and cons

Don’t take our word for it - see why 10 million students trust us with their essay needs.

Tissue Engineering Essay
Tissue Engineering is the science whose object is the physiological regeneration of a biological tissue in laboratory conditions (invitro) and directly in patients (invivo). The physiological regeneration is achieved through the cultivation of the patient's cells inside tissue scaffolds. The scaffolds are the "womb," inside of which the cells multiply and are supported, in order to regenerate tissue while, at the same time, the scaffold is decomposed in non-toxic products. The successful decomposition of the scaffolds, during the development of the tissue, is based on the use of bio-materials, particularly polymers, which share the same attributes in biocompatibility and biodegrability. The tissue scaffolds are manufactured with many methods, and among them are rapid prototyping techniques, that manufacture the scaffolds in layers. The most common among them is the Selective Laser Sintering, SLS), 3D Printing and stereolithography (SLA), which seem to constantly gain ground in the tissue engineering field. …show more content…
The procedure initiates with the study of the object of interest, which is the human skull. Next is the research regarding the conventional methods of restoration and the biomaterials that will be used. From this research, conclusions are drawn regarding the usefulness of the methods of Tissue Engineering. …show more content…
In the end, the objects that were created from the application are presented, and particularly a digital one from the restoration implant. Their usefulness and the conclusions of the procedure are
Ap Biology Lab Essay
The group hypothesized that if worms in a bottle had dirt, compost, oxygen, and a good environment they would survive, reproduce, and improve soil. The groups hypothesis was partially supported by this experiment. For one, most worms did not survive. By the end of the experiment 5 worms remained although the group started out with 27.
Essay On Surgical Technology
Surgical technology is a profession meant for people who don’t visualize their selves working in front of a computer, spending their whole day with paper works or waiting for the time to pass. This line of work suits well for those individuals who are hungry for action. It is a career made for the brave, the fast and the people who consider themselves as OCDs. Surgical technologists are the “worker bees” of the operating room.
Summary Of The Article 'Reprogramming Redux'
I think the author though could've used more facts so she could back up her opinion. Something I learned from this article was that if you culture two different cell lines on a soft substance they can inherit or acquire stem cell like qualities. I think other information that would help me understand this topic better is a deeper explanation on the topic the article gave a brief explanation. Three words that i didn't understand were; progenitors, pluripotent, and PNAS. Progenitors means the parent or creator of something, it did go with the context I was reading.
Jonathan Welham Vocal Case Summary
There were a number of things to keep in mind while attempting to create tissue that could behave like vocal folds.
Embryonic Stem Cell Research Persuasive Essay
Over the years, there have been many controversial issues surrounding medical research, but one of the most arguable topics of all time is the use of embryonic stem cells. Some individuals believe that extracting stem cells from unborn babies will be useful to create new medications or, in most cases, help regenerate damaged cells. Although, many people disagree with the process scientists use to obtain these stem cells. By continuing embryonic stem cell research, scientists are denying an unborn child the chance to live, they are not letting nature take its intended course, and they are not adhering to the religious or moral beliefs of many people.
Biomedical Engineering Pros And Cons
Overall biomedical engineering in general is a thriving technology that will advance the world more than it ever has. As it continues to grow more things have been developed to help human’s live easier and more stable lives. In the future we can look forward to better prosthetic limbs in the area of natural feeling and physical appearance that can help in health and
Essay On Kinesiology
The concept of prosthetics goes further than just making custom fitted artificial limbs for
Persuasive Essay On Xenotransplantation
Zoe Imagine being on a national organ transplant list and have been given a choice. Do you want a human organ or an animal organ? Yes, Xenotransplantation is a large medical breakthrough, but it come with a tremendous amount of risk involved. Animal organs are not meant to be in a humans body. Therefore, human organs should be used for people on the donation list instead of Xenotransplantation.
Intermarriage Resistance In The Books Of Ezra And Nehemiah
Providing a contrast to the intermarriage resistance in the books of Ezra and Nehemiah, Ruth’s story tells a beautiful, however, a different story of a marriage. Customary to this time period, the levirate marriage custom stated if there were no other heirs, a man was required to marry his brother’s widow (Tullock & McEntire, 2012). As a result of the death of Naomi’s husband and her two sons, that were married to Moabite women, Ruth and Orphah, the women were left with no way to preserve their family line (Tullock & McEntire, 2012). In accordance with the persuasion of Naomi, Orphah returned to her home country, however, Ruth vowed to stay with Naomi (Tullock & McEntire, 2012).
Stem Cell Research Persuasive Essay
Globally, scientists are turning to stem cell research as the most promising step to curing many of the harshest diseases and conditions including cancer, Alzheimer’s, stroke, paralysis and many more. Stem cells are useable as a replacement for damaged cells because of their self renewing properties. Their form allows them to act as other types of cells and regenerate as a substitute for the affected cells or as a way of testing new medications. Stroke related disabilities alone account for more than 1.2 million people and millions more are impacted by other cell related disabilities making stem cell research an essential pursuit in order to make strides in medicine (Cunningham 368).
Essay On Vascular Surgery
By Jonas Wilson, Ing. Med. Vascular Surgery The surgical branch dealing with disorders of the circulatory system, which includes arteries, veins and lymphatic vessels, is called vascular surgery.
Essay On The Pros And Cons Of Animal Cloning
Introduction Cloning is the processes that are used in order to generate exact genetic makeup of a cell, tissue, or organism. The term clone refers to the copied material with the same genetic makeup of the original. According to the definition by National Genome Research Institute (NIH) cloning can be differentiated into three types, those are: 1. Gene cloning, which creates copies of genes or segments of DNA. 2.
Essay On Tissue Engineering
Fix or replace damaged tissue; biology has always been the main concern for scientists. Today, the most important instrument for tissue engineering scientists to produce replacement tissues and implants to repair or replace damaged tissue. Tissue engineering is generating a new field of study in which the principles of engineering and biology to correct the damaged tissue, uses and can renewal, operation and maintenance of tissue healing. In order to use an ideal scaffold Tissue engineering should have features such as non-toxic Cell and tissue properties to be fit.
Advanced Dental Technology Essay
This technology allows certified dental technicians to provide restorations on a computer screen, which makes the job easier. The fabricated material can be done in a single appointment and there is no need for temporaries afterwards. Some studies have also demonstrated that CAD/CAM restorations are stronger and less likely to fracture than the milled ones from traditional techniques (Kotrzewski). This type of dental technology is available to produce crowns, veneers, and bridges, which helps to improve oral appearance. The use of the digital impressions disregards some laboratory steps, which leads to a faster and more effective product.
Essay On Human Body
The human body is an amazing thing made up of many different parts. These parts are cells, tissues, organs, and organ systems. For starters, one type of cell makes up one type of tissue. Next, two or more types of tissues make an organ. Then, a few organs working together make an organ system.
More about Tissue Engineering Essay
Related topics.
- Embryonic stem cell
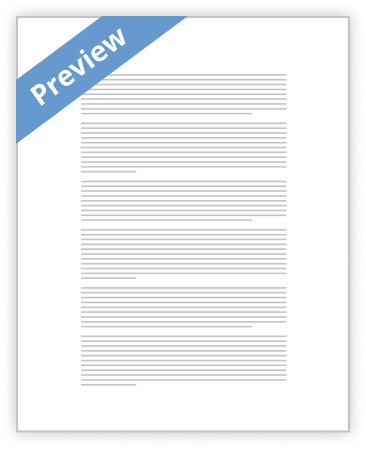
Tissue Engineering Essays
- 3 Works Cited
Tissue Engineering Tissue engineering, labeled by Time.com as the number one hottest job for the 21st century, holds great potential for medicine and the treatment of chronic diseases and disorders. With tissue engineering, familiar problems like the rejection of foreign tissue by the body, the severe shortage of organ donors , and the inefficiency of artificial devices may be solved. However, this cutting edge biotechnology has already spurred intense controversy over the ethics and morality of creating spare human body parts. The goal of tissue engineering is to grow tissues and neo-organs that can be used for transplants. Tissue engineers must first decide what type of cell they want to use and stimulate to grow. Because animal …show more content…
In addition to giving the growing cells a shape to grow into, the scaffold distributes the cells about 2-3 mm apart and allows the cells better access to nutrients and means of waste removal, which is important when trying to grow an evenly distributed functioning organ. For larger, solid organs, such as the liver, pancreas, and kidneys, blood vessels need to be created so the organs have adequate blood supply. By covering the engineered organs with growth factors, angiogenesis, the formation of new vasculature, can be prompted. Although tissue engineers and researchers have already succeeded with creating new skin, blood vessels, bone and cartilage, the more complex organs are difficult to reproduce because of their different functions. Researchers must also be concerned with the mechanisms of growing the tissue. For instance, the advantages and disadvantages are not yet clear for the length of time the cells should be exposed to the growth factors or the difference between growing the tissue outside the body or implanting the scaffold inside the body and letting the tissue grow there. Scaffolding and injectable polymers that form scaffolds in irregular areas (like bone fractures) are also being improved. Much more research is being conducted in order to grow the hearts, livers, breasts, kidneys, and other valuable organs that so many people need. Although tissue engineering has great application for helping ill patients, it
Tissue Engineering in Replace of Organ Donation
Tissue engineering is an emerging interdisciplinary field that uses principles from engineering, biology and chemistry in an effort towards tissue regeneration. The main draw of tissue engineering is the regeneration of a patient’s own tissues and organs free from low biofunctionality and poor biocompatibility and serious immune rejection. As medical care continues to improve and life expectancy continues to grow, organ shortages become more problematic.(Manufacturing living things) According to organdonor.gov, a patient is added to the waiting list every 10 minutes and an average of 18 people die everyday waiting for an organ donation. The “nirvana” of tissue engineering is to replace the need for organ donation altogether. This could be achieved using scaffolding from
3d Printing Case
In 2011, Professor Susmita Bose, of Washington State University, modified a ProMetal 3D printer to bind chemicals to a ceramic powder, creating intricate scaffolds that promote the growth of bone in any shape. Prof. Bose’s goal is to, one day, be able to implant the bone scaffold with bone growth factors in such a way that the implant is dissolved by natural bone material in even load-bearing bone structures.
How Scientists Can Most Efficiently Complete Organ Printing Essay
In the past, the only way to replace diminished cells, tissues, and organs was from organ transplantation. An organ donor was needed, and the tissues would be surgically removed from the donated body and placed into the recipient. Due to the current research being conducted, it is believed that tissue engineering and organ printing can contribute to the process of improving and saving lives.
Anthony Atala's Growing New Organs In TED Talks
I watched Anthony Atala’s speech “Growing new organs” in TED Talks, and was convinced by Anthony that even engineered organ was very a controversial topic, it still brought benefits to patients who need tissue replacement. Anthony’s strongest delivery attribute was his language choices. He opened his speech by showing a story and research statistics of organ transplantation. For example, he pointed out: “every 30 seconds, a patient dies form diseases that could be treated with tissue replacement.” Anthony used the story and number wisely because he caught my attention and brought my interest to his speech. Also, Anthony used examples and stories of his own experience as a surgeon and a researcher. He not only established his credibility, but
Opposing Embryonic Stem Cell Research Essay
Adult stem cells have already proven to be successful in treating diseases and have helped hundreds of thousands of patients, and new clinical uses expand almost weekly. Adult stem cells can be obtained from cord blood, fat, neural tissue, muscle, bone marrow, placental and skin cells. Adult stem cells are increasingly being shown to have a similar and perhaps an identical capacity to become cells of other types. There is a possibility that adult stem cells may function more efficiently and more safely than embryonic cells. Treena Arinzeh, a young professor who last year won a Presidential Award, the nation's highest scientific honor, is bringing the promise of stem cell research one step closer to reality. Adult stem cells also have a unique trait that lends them their magic: Under the right conditions, or given the proper signals, they have the ability to turn into different cell types. Arinzeh is doing exactly that: developing signals, in the form of biomaterials, that will help adult stem cells turn into cells that, if injected into a diseased area of the human body, could regenerate damaged tissue. Her research has also led to two major stem-cell discoveries: One showing that stem cells, when mixed with biomaterials known as scaffolds, can help regenerate bone growth; and another proving that stem cells taken from one person can be successfully implanted into another. A list of conditions for which stem-cell treatment holds promise grows almost daily: It now
Scaffolding Persuasive Speech
This new research uses the scaffolding technique combined with stem cells to grow a heart. The new breakthrough was made using poor quality donated hearts. There were first stripped of heart tissue, leaving only the scaffolding of the heart. All of the tissue must be gone so that a potential patient won't risk rejection.
Essay on “Heaven Scenario.”
Andree, C., et al. “Gene Technology and Tissue Engineering.” Minimally Therapy & Allied Technologies 11.3 (2002): 93-99. Academic Search Premier. Web. 18 March 2014.
Benefits of Cloning vs. Antiquated Religious Beliefs Essay
- 7 Works Cited
Many transplant candidates die while waiting for an organ, whether it be a heart, lung, kidney or liver. Yes, it is true that thousands of people are saved each year by organ transplantation, yet even more die each year waiting while their organs shut down. "In perhaps the most dramatic example, the American Heart Association reports that only 2,300 of 40,000 Americans who needed a new heart in 1997 got one." (Mikos and Mooney 2). The new strategy which seems promising is the development of what Dr. David J. Mooney of the University of Michigan and Dr. Antonios G. Mikos of the M.D. Anderson Cancer Center in Houston call "neo-organs." (3). In one aspiring procedure, the patient receives cells that have been harvested previously and comprised into 3-dimensional molds of biodegradable polymers, such as those used to make dissolvable
Embryonic Stem Cells Argumentative Essay
Because of stem cells regenerative qualities, many scientists hypothesize that eventually we can use stem cells on a large scale to assist us to regenerate damaged tissue within the body especially when transitioning organ donations, or prosthetics into an individual, ultimately making it a safer practice.
Ethical Arguments Against Stem Cells
The medical advances of donor organs and organ transplants have made incredible leaps and bounds in recent decades. Today we can grow stem cells from normal skin tissues, but there are still ethical arguments against the practice of using stem cells for medical procedures. Being able to produce these stem cells from our body tissues is a huge bound forward in medical advances. With that, it is possible to create an organ for the individual who needs it; increasing the organ's viability and ability to be used; ensuring there is no damage or contaminates from donor organs. Now of course even lab-grown organs have its list of ethical backlash that it has faced in recent years. Medical professionals and scientists are already growing organs
Developing A Composite Scaffold That Integrates Growth Factors
In order to develop a scaffold system that significantly improves neural growth, a combination of tissue engineering and regenerative medicine techniques will be executed. First, a composite scaffold that integrates collagen and chitosan, in a 3D porous structure will be fabricated and characterized. Second, the necessary bio-factor composition to promote neural growth in a glial scarring environment will be determined. Third, and lastly, the scaffold’s ability to provide neuroprotection and to biodegrade will be assessed.
Stem Cell Research Essay
- 1 Works Cited
Stem cell research is the future of medical and biological research and remedies, and it is fascinating to watch the progression of this new and important science as it unfolds. These cells were discovered in mouse embryos in the 1980s, and are remarkable because of their potential to grow into a variety of different kinds of cells within a body. Common in fetuses, and more rare in adult animals of all kinds, stem cells can be manipulated in useful ways to repair many tissues, dividing limitlessly for therapeutic purposes. When a stem cell divides, each new cell has the potential either to remain a stem cell or to differentiate into more specialized tissue, such as nerve, pancreas, bone marrow, or unique blood components. Initially
Unwind: Organ Donation After Brain Death
An average of 16 people die in Europe each day without getting the organ they need to survive. 22 people die each day in the United States without getting the organ they need, too. All of these people who die are waiting for organs such like a liver, heart, and other organs that will help keep them alive. The scientists looked at the statistics and wanted to start to develop new organs and body parts to save the patients that are waiting for them. The scientists thought they could start by growing them in a laboratory and make them out of stem cells. They have been struggling with the development of the organs and the progress has been slowed. Other scientists have another theory in how to create a fully functioning organ. They think that everyone should let nature take over and let evolutions happen. Evolutions has helped cells adapt to outside environments and turned our cells into complex molecules that help us survive. The scientists also think that they could use an animal's’ kidney, liver, lungs, heart, and other organs that are useful to keep ourselves alive. Those organs can come from animals, like pigs, because they have somewhat the same kind of organs we have. The only problem that can occur during the operation is that, when you use transfer the heart from a pig, or another animal, our immune system will reject the transplant. People who have been studying about growing human
Organ Transplantation Essay
Lately, there is an emerging innovation whereby organs are created to form and increase in size by a process of inorganic accretion, from the patient’s cell. This field of medicine is known as the regenerative medicine. In addition to this, there are basically various types of regenerative medical
A Research Study On Regenerative Medicine
The basis of tissue engineering involves the production of stem cell, progenitor or precursor cells from embryonic or adult origin, which will induce the regeneration of tissues and organs. The following step is to create biocompatible matrices or scaffolds for the conduction of the signal. These matrices or scaffolds must be able to sustain cell proliferation and differentiation as well as the vascular ingrowth and the unification the new tissue with the surrounding host tissue. Additionally, they must have the capacity to adequately degrade concurrent with tissue regeneration. Then, the induction of growth factors or signalling proteins will activate the proliferation and differentiation of the stem cells. Finally the mechanical stimulation by biochemical forces like shear or strain. Scaffolds can be made in different types of materials like bioceramics, membrane, fibres, foams hydrogels…
Related Topics
- Immune system
- Embryonic stem cells
- Organ donors
An official website of the United States government
Official websites use .gov A .gov website belongs to an official government organization in the United States.
Secure .gov websites use HTTPS A lock ( Lock Locked padlock icon ) or https:// means you've safely connected to the .gov website. Share sensitive information only on official, secure websites.
- Publications
- Account settings
- Advanced Search
- Journal List

Tissue Engineering: Current Strategies and Future Directions
Jennifer l olson, anthony atala, james j yoo.
- Author information
- Article notes
- Copyright and License information
Corresponding Author: James J. Yoo. Wake Forest Institute for Regenerative Medicine, Wake Forest University School of Medicine, Medical Center Boulevard, 1834, Wake Forest Road, Winston-Salem, NC 27109, USA. TEL: +1-336-713-7294, FAX: +1-336-713-7290, [email protected]
Corresponding author.
Received 2011 Mar 31; Accepted 2011 Apr 8; Issue date 2011 Apr.
This is an Open Access article distributed under the terms of the Creative Commons Attribution Non-Commercial License ( http://creativecommons.org/licenses/by-nc/3.0 ) which permits unrestricted non-commercial use, distribution, and reproduction in any medium, provided the original work is properly cited.
Novel therapies resulting from regenerative medicine and tissue engineering technology may offer new hope for patients with injuries, end-stage organ failure, or other clinical issues. Currently, patients with diseased and injured organs are often treated with transplanted organs. However, there is a shortage of donor organs that is worsening yearly as the population ages and as the number of new cases of organ failure increases. Scientists in the field of regenerative medicine and tissue engineering are now applying the principles of cell transplantation, material science, and bioengineering to construct biological substitutes that can restore and maintain normal function in diseased and injured tissues. In addition, the stem cell field is a rapidly advancing part of regenerative medicine, and new discoveries in this field create new options for this type of therapy. For example, new types of stem cells, such as amniotic fluid and placental stem cells that can circumvent the ethical issues associated with embryonic stem cells, have been discovered. The process of therapeutic cloning and the creation of induced pluripotent cells provide still other potential sources of stem cells for cell-based tissue engineering applications. Although stem cells are still in the research phase, some therapies arising from tissue engineering endeavors that make use of autologous, adult cells have already entered the clinical setting, indicating that regenerative medicine holds much promise for the future.
Keywords: Biomaterials, Cell transplantation, Regenerative medicine, Stem cell, Tissue engineering
INTRODUCTION
Patients suffering from diseased and injured organs are often treated with transplanted organs, and this treatment has been in use for over 50 years. In 1955, the kidney became the first entire organ to be replaced in a human, when Murray transplanted this organ between identical twins. Several years later, Murray performed an allogeneic kidney transplant from a non-genetically identical patient into another. This transplant, which overcame the immunologic barrier, marked a new era in medicine and opened the door for use of transplantation as a means of therapy for different organ systems.
As modern medicine increases the human lifespan, the aging population grows, and the need for donor organs grows with it, because aging organs are generally more prone to failure. However, there is now a critical shortage of donor organs, and many patients in need of organs will die while waiting for transplants. In addition, even if an organ becomes available, rejection of organs is still a major problem in transplant patients despite improvements in the methods used for immunosuppression following the transplant procedure. Even if rejection does not occur, the need for lifelong use of immunosuppressive medications leads to a number of complications in these patients.
These problems have led physicians and scientists to look to new fields for alternatives to organ transplantation. In the 1960s, a natural evolution occurred in which researchers began to combine new devices and materials sciences with cell biology, and a new field that is now termed tissue engineering was born. As more scientists from different fields came together with the common goal of tissue replacement, the field of tissue engineering became more formally established. Tissue engineering is now defined as "an interdisciplinary field which applies the principles of engineering and life sciences towards the development of biological substitutes that aim to maintain, restore or improve tissue function." 1 Then, after the discovery of human stem cells by Thomson's group in the early 1980s, 2 the field of stem cell biology took shape and suggested that it may one day be possible to obtain and use donor stem cells in tissue engineering strategies, or perhaps even reactivate endogenous stem cells and use them to regenerate failing organs in adult patients.
The fields of stem cells, cell transplantation, and tissue engineering all have one unifying concept-the regeneration of living tissues and organs. Thus, in 1999, William Haseltine, then the Scientific Founder and Chief Executive Officer of Human Genome Sciences, coined the term regenerative medicine , in effect bringing all these areas under one defining field. 3
In the past two decades, scientists have attempted to engineer virtually every tissue of the human body. This article will review the basic techniques used in tissue engineering and discuss some of the progress that has been achieved in this field.
THE BASIC COMPONENTS OF REGENERATIVE MEDICINE STRATEGIES
The field of regenerative medicine encompasses various areas of technology, such as tissue engineering, stem cells, and cloning. Tissue engineering, one of the major areas of regenerative medicine, follows the principles of cell transplantation, materials science, and engineering toward the development of biological substitutes that can restore and maintain normal function. Tissue engineering strategies generally fall into two categories: the use of acellular scaffolds, which depend on the body's natural ability to regenerate for proper orientation and direction of new tissue growth, and the use of scaffolds seeded with cells. Acellular scaffolds are usually prepared by manufacturing artificial scaffolds or by removing cellular components from tissues via mechanical and chemical manipulation to produce acellular, collagen-rich matrices. 4 - 7 These matrices tend to slowly degrade on implantation and are generally replaced by the extracellular matrix (ECM) proteins that are secreted by the in-growing cells. Cells can also be used for therapy via injection, either with carriers such as hydrogels or alone.
1. Biomaterials for use in regenerative medicine
In the past, synthetic materials were introduced to replace or to rebuild diseased tissues or parts in the human body. The manufacture of new materials, such as tetrafluoroethylene (Teflon) and silicone, opened a new field of research that led to the development of a wide array of devices that could be applied for human use. Although these devices could provide structural support or replacement, the functional component of the original tissue was not restored. However, studies in cell biology, molecular biology, and biochemistry allowed a better understanding of the ECM and its interaction with cells in the tissues of the body, as well as interactions with growth factors and their ligands, and as a result, new biomaterials were designed with these interactions in mind.
In tissue engineering, biomaterials replicate the biological and mechanical function of the native ECM found in tissues in the body. Biomaterials provide a three-dimensional space in which cells can attach, grow, and form new tissues with appropriate structure and function. They also allow for the delivery of cells and appropriate bioactive factors (e.g., cell adhesion peptides, growth factors) to desired sites in the body. 8 Because most mammalian cell types are anchorage-dependent and will die if no cell-adhesion substrate is available, biomaterials provide this substrate while allowing delivery of cells with high loading efficiency. Biomaterials can also provide mechanical support against in vivo forces so that the predefined three-dimensional structure of a tissue-engineered organ is maintained during tissue development.
The ideal biomaterial should be biodegradable and bioresorbable to support the replacement of normal tissue without inducing inflammation. Incompatible materials are destined for an inflammatory or foreign-body response that eventually leads to rejection or necrosis. Because biomaterials provide temporary mechanical support while the cells undergo spatial reorganization into tissue, a properly chosen biomaterial should allow the engineered tissue to maintain sufficient mechanical integrity to support itself in early development, while in late development, it should have begun degradation such that it does not hinder further tissue growth. 8 The degradation products, if produced, should be removed from the body via metabolic pathways at an adequate rate to ensure that the concentration of these degradation products in the tissues remains at a tolerable level. 9
Generally, three classes of biomaterials have been utilized for engineering tissues: naturally derived materials (e.g., collagen and alginate), 10 - 14 acellular tissue matrices (e.g., bladder submucosa and small intestinal submucosa), 4 - 7 and synthetic polymers such as polyglycolic acid (PGA), polylactic acid (PLA), and poly (lactic-co-glycolic acid) (PLGA). 15 - 18 These classes of biomaterials have been tested with respect to their biocompatibility. 19 , 20 Naturally derived materials and acellular tissue matrices have the potential advantage of biological recognition. However, synthetic polymers can be produced reproducibly on a large scale with controlled properties such as strength, degradation rate, and microstructure.
2. Cells for use in cell therapy and tissue engineering
1) native cells.
When native cells are used for tissue engineering, a small piece of donor tissue is dissociated into individual cells. These cells are expanded in culture and either injected directly back into the host or attached to a support matrix and then reimplanted. The source of donor tissue can be heterologous (such as bovine), allogeneic (same species, different individual), or autologous. The preferred cells to use are autologous cells, where a biopsy of tissue is obtained from the host, the cells are dissociated and expanded in culture, and the expanded cells are implanted into the same host. 5 , 21 - 28 The use of autologous cells, although it may cause an inflammatory response, avoids rejection, and thus the deleterious side effects of immunosuppressive medications can be avoided.
Ideally, both structural and functional tissue replacement will occur with minimal complications when autologous native cells are used. However, one of the limitations of applying cell-based regenerative medicine techniques to organ replacement has been the inherent difficulty of growing specific cell types in large quantities. Even when some organs, such as the liver, have a high regenerative capacity in vivo , cell growth and expansion in vitro may be difficult. By studying the privileged sites for committed precursor cells in specific organs, as well as exploring the conditions that promote differentiation, one may be able to overcome the obstacles that limit cell expansion in vitro . For example, urothelial cells could be grown in the laboratory setting in the past, but only with limited expansion. Several protocols were developed over the past two decades that identified the undifferentiated cells and kept them undifferentiated during their growth phase. 27 , 29 , 30 - 32 With the use of these methods of cell culture, it is now possible to expand a urothelial strain from a single specimen that initially covered a surface area of 1 cm 2 to one covering a surface area of 4,202 m 2 (the equivalent of one football field) within 8 weeks. 27 These studies indicated that it should be possible to collect autologous bladder cells from human patients, expand them in culture, and return them to the donor in sufficient quantities for reconstructive purposes. 27 , 30 Major advances have been achieved within the past decade on the possible expansion of a variety of primary human cells, with specific techniques that make the use of autologous cells for clinical application possible.
Most current strategies for tissue engineering depend on a sample of autologous cells from the diseased organ of the host. However, for many patients with extensive end-stage organ failure, a tissue biopsy may not yield enough normal cells for expansion and transplantation. In other instances, primary autologous human cells cannot be expanded from a particular organ, such as the pancreas. In these situations, stem cells are envisioned as being an alternative source of cells from which the desired tissue can be derived. Stem cells can be derived from discarded human embryos (human embryonic stem cells), from fetal tissue, or from adult sources (bone marrow, fat, skin).
3. Stem cells for use in tissue engineering
1) embryonic stem cells.
Human embryonic stem (hES) cells exhibit two remarkable properties: the ability to proliferate in an undifferentiated but pluripotent state (self-renewal), and the ability to differentiate into many specialized cell types. 36 They can be isolated by aspirating the inner cell mass from the embryo during the blastocyst stage (5 days post-fertilization) and are usually grown on feeder layers consisting of mouse embryonic fibroblasts or human feeder cells. 37 More recent reports have shown that these cells can be grown without the use of a feeder layer 38 and thus avoid the exposure of these human cells to mouse viruses and proteins. These cells have demonstrated longevity in culture by maintaining their undifferentiated state for at least 80 passages when grown by use of current published protocols. 2 , 39 In addition, hES cells are able to differentiate into cells from all three embryonic germ layers in vitro . Skin and neurons have been formed, indicating ectodermal differentiation. 40 - 43 Blood, cardiac cells, cartilage, endothelial cells, and muscle have been formed, indicating mesodermal differentiation. 44 - 46 Pancreatic cells have been formed, indicating endodermal differentiation. 47 In addition, as further evidence of their pluripotency, embryonic stem cells can form embryoid bodies, which are cell aggregations that contain all three embryonic germ layers while in culture and can form teratomas in vivo . 48 However, there are many ethical and religious concerns associated with hES cells because embryos are destroyed in order to obtain them. Thus, the use of these cells is currently banned in many countries.
2) Stem cells from somatic cell nuclear transfer
Stem cells for tissue engineering could also be generated through cloning procedures. There has been tremendous interest in the field of nuclear cloning since the birth of the cloned sheep Dolly in 1997, but actually, Dolly was not the first animal produced by using nuclear transfer. In fact, frogs were the first successfully cloned vertebrates derived from nuclear transfer. 49 However, in the frog experiment, the nuclei used for cloning were derived from non-adult sources. In fact, live lambs were produced in 1996 by using nuclear transfer as well, but they were produced from differentiated epithelial cells derived from embryonic discs. 50 The significance of Dolly was that she was the first mammal to be derived from an adult somatic cell by use of nuclear transfer. 51 Since then, animals from several species have been grown by using nuclear transfer technology, including cattle, 52 goats, 53 mice, 54 and pigs. 55 - 56
Two types of nuclear cloning, reproductive cloning and therapeutic cloning, have been described, and a better understanding of the differences between the two types may help to alleviate some of the controversy that surrounds these technologies. 57 - 58 Banned in most countries for human applications, reproductive cloning is used to generate an embryo that has the identical genetic material as its cell source. This embryo can then be implanted into the uterus of a female to give rise to an infant that is a clone of the donor. On the other hand, therapeutic cloning is used to generate early stage embryos that are explanted in culture to produce embryonic stem cell lines whose genetic material is identical to that of its source. These autologous stem cells have the potential to become almost any type of cell in the adult body, and thus would be useful in tissue and organ replacement applications. 59 Therefore, therapeutic cloning, which has also been called somatic cell nuclear transfer, may provide an alternative source of transplantable cells. According to data from the Centers for Disease Control and Prevention, an estimated 3,000 Americans die every day of diseases that could have been treated with stem cell-derived tissues. 60 - 61 With current allogeneic tissue transplantation protocols, rejection is a frequent complication because of immunologic incompatibility, and immunosuppressive drugs are usually required. 59 The use of transplantable tissue and organs derived from therapeutic cloning could lead to the avoidance of immune responses that typically are associated with transplantation of non-autologous tissues. 60
While promising, somatic cell nuclear transfer technology has certain limitations that require further study before this technique can be applied widely in tissue or organ replacement therapy. First, the efficiency of the cloning process is very low, as evidenced by the fact that most embryos derived from the cloning process do not survive. 62 - 64 To improve cloning efficiency, further improvements are required in many of the complex steps of nuclear transfer, such as the enucleation process for oocytes, the actual transfer of a nucleus to this enucleated oocyte, and the activation process that instructs the cloned oocytes to begin dividing. In addition, cell cycle synchronization between donor cells and recipient oocytes must be accomplished. 65
3) Reprogramming and generation of iPS cells
Within the past few years, exciting reports of the successful transformation of adult somatic cells into pluripotent stem cells through genetic "reprogramming" have been published. Reprogramming is a technique that involves de-differentiation of adult somatic cells (such as fibroblasts) to produce patient-specific pluripotent stem cells. This process is especially exciting because it allows pluripotent stem cells to be obtained without the use of embryos. Also, cells generated by reprogramming are genetically identical to the somatic cells used (and thus to the patient who donated these cells) and should not be rejected. Yamanaka was the first to discover that mouse embryonic fibroblasts (MEFs) and adult mouse fibroblasts could be reprogrammed into an "induced pluripotent state (iPS)." 66 They examined 24 genes that were thought to be important for embryonic stem cells and identified 4 key genes that, when introduced into the reporter fibroblasts via retroviral vectors, resulted in drug-resistant cells. These were Oct3/4 , Sox2 , c-Myc , and Klf4 . The resultant iPS cells possessed the immortal growth characteristics of self-renewing embryonic stem cells, expressed genes specific for embryonic stem cells, and generated embryoid bodies in vitro and teratomas in vivo . When iPS cells were injected into mouse blastocysts, they contributed to a variety of cell types. However, although iPS cells selected in this way were pluripotent, they were not identical to embryonic stem cells. Unlike embryonic stem cells, chimeras made from iPS cells did not result in full-term pregnancies. Gene expression profiles of the iPS cells showed that they possessed a distinct gene expression signature that was different from that of embryonic stem cells. In addition, the epigenetic state of the iPS cells was somewhere between that found in somatic cells and that found in embryonic stem cells, suggesting that the reprogramming was incomplete.
These results were improved significantly by Wernig and Jaenisch in July 2007. 67 Fibroblasts were infected with retroviral vectors and selected for the activation of endogenous Oct4 or Nanog genes. Results from this study showed that DNA methylation, gene expression profiles, and the chromatin state of the reprogrammed cells were similar to those of embryonic stem cells. Teratomas induced by these cells contained differentiated cell types representing all three embryonic germ layers. Most importantly, the reprogrammed cells from this experiment could form viable chimeras and contribute to the germline-like embryonic stem cells, suggesting that these iPS cells were completely reprogrammed. Wernig et al observed that the number of reprogrammed colonies increased when drug selection was initiated later (day 20 rather than day 3 post-transduction). This suggests that reprogramming is a slow and gradual process and may explain why previous attempts resulted in incomplete reprogramming.
It has recently been shown that reprogramming of human cells is possible. 68 - 69 Yamanaka generated human iPS cells that are similar to hES cells in terms of morphology, proliferation, gene expression, surface markers, and teratoma formation. Thompson's group showed that retroviral transduction of the stem cell markers OCT4 , SOX2 , NANOG , and LIN28 could generate pluripotent stem cells. However, in both studies, the human iPS cells were similar but not identical to hES cells. Although reprogramming is an exciting phenomenon, our limited understanding of the mechanism underlying it currently limits the clinical applicability of the technique, but the future potential of reprogramming is quite exciting.
4) Amniotic fluid and placental stem cells
An alternate source of stem cells is the amniotic fluid and placenta. Amniotic fluid and the placenta are known to contain multiple partially differentiated cell types derived from the developing fetus. We isolated stem cell populations from these sources, called amniotic fluid and placental stem cells (AFPSC), that express embryonic and adult stem cell markers. 70 The undifferentiated stem cells expand extensively without feeders and double every 36 hours. Unlike hES cells, the AFPSC do not form tumors in vivo . Lines maintained for over 250 population doublings retained long telomeres and a normal karyotype. AFS cells are broadly multipotent. Clonal human lines verified by retroviral marking can be induced to differentiate into cell types representing each embryonic germ layer, including cells of adipogenic, osteogenic, myogenic, endothelial, neuronal, and hepatic lineages. In this respect, they meet a commonly accepted criterion for pluripotent stem cells, without implying that they can generate every adult tissue. Examples of differentiated cells derived from AFS cells and displaying specialized functions include neuronal lineage cells secreting the neurotransmitter L-glutamate or expressing G-proteingated inwardly rectifying potassium (GIRK) channels, hepatic lineage cells producing urea, and osteogenic lineage cells forming tissue engineered bone. The cells could be obtained either from amniocentesis or chorionic villous sampling in the developing fetus, or from the placenta at the time of birth. The cells could be preserved for self-use and used without rejection, or they could be banked. A bank of 100,000 specimens could potentially supply 99% of the US population with a perfect genetic match for transplantation. Such a bank may be easier to create than with other cell sources, because there are approximately 4.5 million births per year in the USA. 70
5) Adult stem cells
Adult stem cells, especially hematopoietic stem cells, are the best understood cell type in stem cell biology. 71 The presence of stem cells in the adult was first discerned by Till and McCulloch, who were investigating the mechanisms by which the bone marrow could regenerate after exposure to radiation. 72 However, adult stem cell research remains an area of intense study, because their potential for therapy may be applicable to a myriad of degenerative disorders. Within the past decade, adult stem cell populations have been found in many adult tissues other than the bone marrow and the gastrointestinal tract, including the brain, 73 - 74 skin, 75 and muscle. 76 Many other types of adult stem cells have been identified in organs all over the body and are thought to serve as the primary repair entities for their corresponding organs. 77 The discovery of such tissue-specific progenitors has opened up new avenues for research.
A notable exception to the tissue-specificity of adult stem cells is the mesenchymal stem cell (MSC), also known as the multipotent adult progenitor cell. This cell type is derived from bone marrow stroma. 78 - 79 Such cells can differentiate in vitro into numerous tissue types 80 - 81 and can also differentiate developmentally if injected into a blastocyst. Multipotent adult progenitor cells can develop into a variety of tissues including neuronal, 82 adipose, 76 muscle, 76 , 83 liver, 84 - 85 lungs, 86 spleen, 87 and gut tissue, 79 but notably not bone marrow or gonads.
In addition, stem cells derived from adipose tissue may also be an autologous and self-renewing cell source. Adipose-derived stem cells (ADSCs) have been shown to differentiate into a variety of cell phenotypes, and since they are easily obtained, they show great promise for future types of reconstructive surgery based on tissue engineering and there have been several clinical trials using these cells. Wilson and Mizuno have both provided excellent, detailed reviews of these. 88 - 89
Research into more differentiated types of adult stem cells has, however, progressed slowly, mainly because investigators have had great difficulty in maintaining adult non-mesenchymal stem cells in culture. Some cells, such as those of the liver, pancreas, and nerve, have very low proliferative capacity in vitro , and the functionality of some cell types is reduced after the cells are cultivated. Isolation of cells has also been problematic, because stem cells are present in extremely low numbers in adult tissue. 84 , 90 While the clinical utility of adult stem cells is currently limited, great potential exists for future use of such cells in tissue-specific regenerative therapies. The advantage of adult stem cells is that they can be used in autologous therapies, thus avoiding any complications associated with immune rejection.
CELLULAR THERAPIES
The simplest regenerative medicine strategies are those that are based on the actions of cells, which can be implanted either alone or within a type of carrier material, such as a hydrogel. These cell therapies are designed to inject or implant healthy cells to replace populations of cells that are no longer functioning properly owing to disease or injury. The cells used in these therapies can be autologous cells derived from a tissue biopsy and expanded in culture, or they can be stem cells from various sources that can be guided to differentiate into appropriate cell types by using both endogenous and exogenous biochemical cues.
For example, one area of intense study in regenerative medicine is the pancreas, because the ability to replace or regenerate the insulin-producing cells of this organ could lead to novel treatments or a cure for diabetes. In a series of exciting experiments, Zhou et al demonstrated that regeneration of the insulin-producing cells of the pancreas, the β-cells, may be possible by using cellular reprogramming techniques 91 Using a mouse model, they showed that in vivo activation of a specific combination of three transcription factors (Ngn3, Pdx1, and Mafa) by use of adenoviral vectors led to the reprogramming of adult differentiated pancreatic exocrine cells into cells that closely resembled β-cells. These cells were similar to native β-cells in size, shape, and ultrastructure, and they expressed genes that are specific to β-cells as well. Interestingly, these cells secreted insulin and expressed vascular endothelial growth factor (VEGF), which allowed them to remodel the local vasculature in a manner similar to native β-cells. In fact, these reprogrammed cells were able to partially ameliorate hyperglycemia in diabetic mice, suggesting that reprogramming techniques for treating disease may one day become a reality.
Degenerative muscle diseases such as Duchenne's muscular dystrophy have devastating effects on quality of life. To date, these genetic disorders have no suitable treatment. Early enthusiasm for gene therapy interventions has been tempered by issues of vector toxicity and inadequate gene transfer to target muscle cells in vivo . However, natural mechanisms of muscle repair have suggested that cell-based therapy could take advantage of natural homing mechanisms to direct cells to the proper location. 92 Experiments using the mdx mouse model, in which the dystrophin gene is mutated, indicate that injection of normal muscle precursors and dermal fibroblasts into skeletal muscle can lead to increased expression of dystrophin and improved functional outcomes. However, this treatment option requires further studies before it can be widely applied in the clinic.
Although many of these cell therapies are still in the experimental stage, some are being translated to the clinic and clinical trials are being performed. Vesicoureteral reflux (VUR; a condition in which urine flows backwards from the bladder into the ureter and kidney) and stress urinary incontinence are two urologic conditions that can result from dysfunction of a specific sphincter muscle. When severe, these conditions are repaired surgically. However, cell-based therapies for both VUR and incontinence would be an important alternative to surgical repair of these conditions. Ideally, such a therapy would be easily administered by injection and well tolerated by the patient. The injectable therapy should be non-antigenic, non-migratory, volume stable, and safe for human use, and in addition, it should be able to carry cells and serve as a matrix in vivo .
Toward this goal, long-term studies were conducted to determine the effects of injectable chondrocytes for the treatment of VUR in vivo . 93 Chondrocytes were chosen because the use of autologous cartilage for the treatment of VUR in humans would satisfy all of the requirements for an ideal injectable cell-based therapy. Chondrocytes derived from an ear biopsy can be readily grown and expanded in culture. Neocartilage formation can be achieved in vitro and in vivo by using chondrocytes cultured on synthetic biodegradable polymers. In the VUR experiments, chondrocytes were suspended in an alginate matrix and injected around the vesicoureteral sphincter. In time, normal cartilage replaced the alginate as the alginate slowly degraded. This system was then adapted for the treatment of VUR in a porcine model. 94 These studies show that chondrocytes can be easily harvested and combined with alginate in vitro , that the suspension can be easily injected cystoscopically, and that the elastic cartilage tissue formed can correct the VUR without any evidence of obstruction.
Two multicenter clinical trials were conducted by use of this engineered chondrocyte technology. First, patients with VUR were treated at 10 centers throughout the United States. The patients had a similar success rate as with other injectable substances in terms of cure. Cartilage formation was not noted in patients with treatment failure. Patients who were cured probably had a biocompatible region of engineered autologous tissue present. 95 Secondly, patients with urinary incontinence were treated endoscopically with injected chondrocytes at three different medical centers. Phase 1 trials showed an approximate success rate of 80% at 3 and 12 months postoperatively. 96
TISSUE THERAPIES
Tissue engineering strategies are often referred to as "growing organs in the laboratory." In these strategies, differentiated cells or stem cells are seeded onto a biomaterial scaffold and this construct is allowed to mature in vitro in a bioreactor for a short time before implantation in vivo . These constructs are designed to replace a malfunctioning organ in its entirety. In recent years, it has been shown that hollow organs, such as the urinary bladder, urethra, and blood vessels, can be successfully engineered in the laboratory, and these successes are described below.
The urethra can be repaired by using tissue-engineered grafts in several ways. It has been shown that various biomaterials without cells, such as PGA and acellular collagen-based matrices from small intestine and bladder, can be used experimentally (in animal models) for the regeneration of urethral tissue. 7 , 97 - 99 Acellular collagen matrices derived from bladder submucosa have been used experimentally and clinically. In animal studies, segments of the urethra were resected and replaced with acellular matrix grafts in an onlay fashion. Histological examination showed complete epithelialization and progressive vessel and muscle infiltration, and the animals were able to void through the neo-urethras. 7 These results were confirmed in a clinical study of patients with hypospadias and urethral stricture disease. 100 Decellularized cadaveric bladder submucosa was used as an onlay matrix for urethral repair in patients with stricture disease and hypospadias. Patent, functional neo-urethras were noted in these patients with up to a 7-year follow-up. The use of an off-the-shelf matrix appears to be beneficial for patients with abnormal urethral conditions and obviates the need for obtaining autologous grafts, thus decreasing operative time and eliminating donor site morbidity.
Unfortunately, the above techniques are not applicable for tubularized urethral repairs. The collagen matrices are able to replace urethral segments only when used in an onlay fashion. However, if a tubularized repair is needed, the collagen matrices should be seeded with autologous cells to avoid the risk of stricture formation and poor tissue development. 101 In addition, cell-seeded matrices must be used if the segment of urethra to be replaced is longer than about 1 cm. 102 Recently, Raya-Rivera and colleagues used tissue-engineered urethras that had been created from patients' own cells for tubularized urethral reconstruction. In this preliminary study, five boys who had urethral defects were treated. A tissue biopsy was taken from each patient, and the muscle and epithelial cells derived from the biopsy sample were expanded and seeded onto tubularized polyglycolic acid:poly(lactide-co-glycolide acid) scaffolds to create neo-urethras for implantation. The patients then underwent urethral reconstruction with the engineered urethras. After surgery, these patients were followed for up to 6 years. All of the patients experienced an increase in urinary flow rate, and serial radiographic and endoscopic studies showed that they maintained wide urethral calibers throughout the follow-up period and did not develop strictures. In addition, urethral biopsies were performed in these patients, and these revealed that by 3 months post-surgery, the engineered grafts had developed a normal appearing tissue architecture consisting of a urothelial layer surrounded by a muscular layer. 103
In addition, similar techniques have been used to create tissue-engineered bladder constructs. Urothelial and muscle cells can be expanded in vitro , seeded onto polymer scaffolds, and allowed to attach and form sheets of cells. 104 These principles were applied in several studies in which tissue-engineered bladders were used to repair subtotal cystectomies in beagle dogs. 28 , 105 - 106 The first clinical experience in which engineered bladder tissue for cystoplasty reconstruction in patients was conducted starting in 1999. A small pilot study of seven patients was reported, using a collagen scaffold seeded with cells either with or without omentum coverage, or a combined PGA-collagen scaffold seeded with cells and omental coverage. The patients reconstructed with the engineered bladder tissue created with the PGA-collagen cell-seeded scaffolds showed increased compliance, decreased end-filling pressures, increased capacities, and longer dry periods. 107 Although the experience is promising in terms of showing that engineered tissues can be implanted safely, it is just a start in terms of accomplishing the goal of engineering fully functional bladders. Further experimental and clinical work is being conducted.
Xenogenic or synthetic materials have been used as replacement blood vessels for complex cardiovascular lesions. However, these materials typically lack growth potential, and may place the recipient at risk for complications such as stenosis, thromboembolization, or infection. 108 Tissue-engineered vascular grafts have been constructed by using autologous cells and biodegradable scaffolds and have been applied in dog and lamb models. 109 - 112 The key advantage of using these autografts is that they degrade in vivo and thus allow the new tissue to form without the long-term presence of foreign material. 108 Translation of these techniques from the laboratory to the clinical setting has begun, with autologous vascular cells harvested, expanded, and seeded onto a biodegradable scaffold. 113 The resultant autologous construct was used to replace a stenosed pulmonary artery that had been previously repaired. Seven months after implantation, no evidence of graft occlusion or aneurysmal changes was noted in the recipient. In addition, another group created tissue-engineered blood vessels by using the cell-sheet multilayer method and then used these constructs to successfully create vascular access points for hemodialysis in 10 patients. 114 More recently, the same group completed a larger study on these engineered vessels for hemodialysis, which indicated that the 1-month and 6-month patency of the grafts was 78% and 60%, respectively, which meets the approved criteria for a high-risk patient cohort. 115
Finally, few treatment options are currently available for patients who suffer from severe congenital tracheal pathology, such as stenosis, atresia, and agenesis, due to the limited availability of autologous transplantable tissue in the neonatal period. Tissue engineering in the fetal period may be a viable alternative for the surgical treatment of these prenatally diagnosed congenital anomalies, because cells could be harvested and grown into transplantable tissue in parallel with the remainder of gestation. Chondrocytes from both elastic and hyaline cartilage specimens have been harvested from fetal lambs, expanded in vitro , and then dynamically seeded onto biodegradable scaffolds. 116 The constructs were then implanted as replacement tracheal tissue in fetal lambs. The resultant tissue-engineered cartilage was noted to undergo engraftment and epithelialization, while maintaining its structural support and patency.
Recently, Martin Birchall's group moved this technology into a human patient with end-stage airway disease. 117 This group was able to remove the cellular material and MHC antigens from a human donor trachea and, using a specialized bioreactor, seed this acellular matrix with chondrocytes and epithelial cells derived from the patient to receive the graft. This construct was then used to replace the patient's left main bronchus. There were no perioperative complications, and the left lung ventilated normally as soon as the graft was placed. At 3 months after surgery, the patient's lung function was in the normal range for her age and sex, and she was able to function normally. Although longer follow-up and larger study populations are needed, this report indicates that tissue engineering may be a new option for patients with airway disease.
However, whereas there has been exciting progress with tissue engineering techniques for hollow organs, the development of methods to generate larger, solid organs with more complex histological structure has been much more difficult. A number of issues must be addressed before fully functional, engineered organs such as liver and kidney can be prepared in the laboratory. First, these organs contain extremely complex internal structures made up of numerous cell types arranged in very specific ways, and simple cell-seeding techniques may not be sufficient for reconstructing these structures. In addition, the large size of these organs dictates that the delivery of oxygen and nutrients to each part of the organ will be a challenge, unless a method for engineering a functional vascular network within the organ can be found. However, despite the challenges, there have been some encouraging results from several studies. For example, the kidney contains multiple cell types and a complex functional anatomy that renders it one of the most difficult to reconstruct, 21 , 118 yet we were able to create a rudimentary form of this organ that appeared to have at least the filtration properties of the native kidney.
We applied the principles of both tissue engineering and therapeutic cloning in an effort to produce genetically identical renal tissue in a large animal model, the cow ( Bos taurus ). 119 Bovine skin fibroblasts from adult Holstein steers were obtained by ear notch, and single donor cells were isolated and microinjected into the perivitelline space of donor enucleated oocytes (nuclear transfer). The resulting blastocysts were implanted into progestin-synchronized recipients to allow for further in vivo growth. After 12 weeks, cloned renal cells were harvested and expanded in vitro . Next, the cloned renal cells were seeded on scaffolds consisting of three collagen-coated cylindrical silastic catheters. The ends of the three membranes of each scaffold were connected to catheters that terminated into a collecting reservoir. This created a renal neo-organ with a mechanism for collecting the excreted urinary fluid. These scaffolds with the collecting devices were transplanted subcutaneously into the same steer from which the genetic material originated and then retrieved 12 weeks after implantation.
At this time, a yellow urine-like fluid was observed collecting within the reservoir of the device. Chemical analysis of this fluid, including urea nitrogen and creatinine levels, electrolyte levels, specific gravity, and glucose concentration, revealed that the implanted renal cells possessed filtration, reabsorption, and secretory capabilities. Histological examination of the retrieved implants revealed extensive vascularization and self-organization of the cells into glomeruli and tubule-like structures. A clear continuity between the glomeruli, the tubules, and the silastic catheter was noted that allowed the passage of urine into the collecting reservoir. These studies demonstrated that cells derived from nuclear transfer can be successfully harvested, expanded in culture, and transplanted in vivo with the use of biodegradable scaffolds on which the single suspended cells can organize into tissue structures that are genetically identical to those of the host. These studies were the first demonstration of the use of therapeutic cloning for regeneration of tissues in vivo . However, the size of this device was small, and the challenge will be to create a larger device with functioning vasculature and innervations, so that it can replace all of the myriad metabolic functions of the kidney.
CHALLENGES AND FUTURE DIRECTIONS IN REGENERATIVE MEDICINE: TRANSLATING REGENERATIVE THERAPIES TO THE CLINIC
The experiences with urethral, bladder, blood vessel, and tracheal replacement using tissue engineering provide encouragement for future efforts to engineer other organs in the laboratory. These experiences also cast light on unsolved problems. For example, innervation of tissues and organs is important for achieving full functionality. In the canine engineered bladder experiments, the observation of positive S-100 staining was consistent with growth of neural structures into the neo-bladders, and bladder function was restored soon after implantation. 28 Innervation of tissue-engineered constructs has been observed in other systems such as the small intestine. 120 Not only is successful connection with the nervous system important for the functionality of neo-organs, but evidence suggests that it can enhance tissue regeneration. 121 - 122 The controlled release of neurotrophic factors is one potential approach to promote peripheral nerve regeneration and synapse formation with engineered tissue. 123 Direct electrical stimulation has proven useful in muscle regeneration 124 and may have broader applicability.
An even more fundamental issue for the ultimate success of laboratory-grown organs, particularly those with complex three-dimensional structure, is the provision of adequate oxygen and the generation of new vasculature. It has been appreciated for some years that in metabolically active tissues, the distance over which oxygen typically must diffuse from a capillary bed to reach a cell is about 0.1 mm, but that in clinical grafts, the distance from the edge to the center of the graft is likely to exceed that by at least 50-fold. 125 - 126 Therefore, with few exceptions (e.g., cartilage), oxygen is rate-limiting for the viability of grafted cells, and thus for organ engineering. Neovascularization, an intricate morphogenetic process that allows the formation of extensively branched vessels, even in an adult, must occur rapidly and efficiently for a grafted neo-organ to thrive after implantation. 127 Moreover, special measures may be necessary to ensure survival of grafted tissue during the initial period after implantation, until a functional vascular bed is in place. Currently, three types of strategies have been devised to solve the oxygen supply problem.
The first strategy involves the use of mechanical or chemical sources of oxygen that can support the construct before and immediately after implantation, until the neovascularization process is completed and can provide the neo-organ with sufficient blood circulation. An intra-tissue perfusion system utilizing an array of micro-needles to deliver oxygen and nutrients and eliminate waste enhances the viability and functionality of thick (1 mm) slices of liver tissue in vitro and might facilitate in vivo grafting. 128 In addition, the use of oxygen-carrying molecules such as perfluorocarbons could promote the function of cells in culture and of encapsulated cells and organ constructs implanted into animals. 129 - 130 Our laboratory recently showed that a PLGA film incorporating an oxygen-generating system (sodium percarbonate) could prevent the necrosis of ischemic tissue over several days in vivo . 131 We hope to develop such novel scaffold materials further to support the survival of large, complex organ constructs in the initial period after implantation.
Second, "prevascularization" strategies aim to generate neo-organs engineered with a preexisting channel structure to facilitate the generation of a competent vascular network. 130 , 132 To accomplish this, endothelial lineage cells can be pre-seeded into the channels or may be recruited in vivo by using biochemical signals that are embedded in or released by the scaffold. However, there is still the question of how to create channels in a way that will be interpreted as a natural vascular network by the body. One solution would be to employ decellularized tissue as the scaffold. A recent study demonstrated that perfusion of an entire heart with detergents yields an acellular structure in which the native vascular channels remain intact. 133 We independently devised perfusion-decellularization technology using liver tissue and have found that the vascular tree of the whole organ scaffold remains patent and can be repopulated with large numbers of endothelial cells. 134 Alternatively, several technologies can be used to manufacture scaffolds with preformed channels, potentially with cells incorporated, designed to promote neo-vascularization. For example, laser guided "writing" was used to pattern endothelial cells and promote their aggregation into tubular vessels. 135 Similarly, ink-jet-based bioprinting of cells and biomaterials by thermal ink jet technology can provide remarkable control of the fine structure of engineered tissues, including the generation of intricate vessel networks. 136 We have used layer-by-layer ink jet printing to produce three-dimensional constructs containing endothelial cells and showed that these develop functional microvascularization when implanted in vivo , as assessed by magnetic resonance imaging. 137 Electrospinning of living cells with biomaterials offers similar potential to fabricate organ structures with pre-patterned vessels. 138 Mathematical modeling of scaffolds designed to contain a preexisting arteriovenous loop shows how the provision of an oxygen source within the scaffold can dynamically support further neo-vascularization and tissue development. 139
Third, it is well established that growth factors such as VEGF and FGF can promote vascularization in engineered tissues. 140 Recent efforts have extended this approach by incorporating additional pro-angiogenic molecules into scaffolds, such as organ-specific ECM from liver to support sinusoidal endothelial cells. 141 Synthetic biomaterials designed to provide signals normally presented by the ECM will complement, and may eventually supersede, the use of the native molecules. 142
Finally, several of the clinical trials involving bioengineered products have been placed on hold because of the costs involved with the specific technology. With a bioengineered product, costs are usually high because of the biological nature of the therapies involved, and as with any therapy, the cost that the medical health care system can allow for a specific technology is limited. Therefore, the costs of bioengineered products have to be lowered before they can have an impact clinically. This is currently being addressed for multiple tissue-engineered technologies. As the technologies advance over time, and the volume of the application is considered, costs will naturally decrease.
SUMMARY AND CONCLUSION
Regenerative medicine efforts are currently underway experimentally for virtually every type of tissue and organ within the human body. As regenerative medicine incorporates the fields of tissue engineering, cell biology, nuclear transfer, and materials science, personnel who have mastered the techniques of cell harvest, culture, expansion, transplantation, and polymer design are essential for the successful application of these technologies to extend human life. Various tissues are at different stages of development, with some already being used clinically, a few in preclinical trials, and some in the discovery stage. Recent progress suggests that engineered tissues may have an expanded clinical applicability in the future and may represent a viable therapeutic option for those who would benefit from the life-extending benefits of tissue replacement or repair.
- 1. Atala A, Lanza RP. Preface. In: Atala A, Lanza RP, editors. Methods of tissue engineering. San Diego: Academic Press; 2001. [ Google Scholar ]
- 2. Thomson JA, Itskovitz-Eldor J, Shapiro SS, Waknitz MA, Swiergiel JJ, Marshall VS, et al. Embryonic stem cell lines derived from human blastocysts. Science. 1998;282:1145–1147. doi: 10.1126/science.282.5391.1145. [ DOI ] [ PubMed ] [ Google Scholar ]
- 3. Hasetine W. A brave new medicine. A conversation with William Haseltine. Interview by Joe Flower. Health Forum J. 1999;42:28–30. [ PubMed ] [ Google Scholar ]
- 4. Dahms SE, Piechota HJ, Dahiya R, Lue TF, Tanagho EA. Composition and biomechanical properties of the bladder acellular matrix graft: comparative analysis in rat, pig and human. Br J Urol. 1998;82:411–419. doi: 10.1046/j.1464-410x.1998.00748.x. [ DOI ] [ PubMed ] [ Google Scholar ]
- 5. Yoo JJ, Meng J, Oberpenning F, Atala A. Bladder augmentation using allogenic bladder submucosa seeded with cells. Urology. 1998;51:221–225. doi: 10.1016/s0090-4295(97)00644-4. [ DOI ] [ PubMed ] [ Google Scholar ]
- 6. Piechota HJ, Dahms SE, Nunes LS, Dahiya R, Lue TF, Tanagho EA. In vitro functional properties of the rat bladder regenerated by the bladder acellular matrix graft. J Urol. 1998;159:1717–1724. doi: 10.1097/00005392-199805000-00100. [ DOI ] [ PMC free article ] [ PubMed ] [ Google Scholar ]
- 7. Chen F, Yoo JJ, Atala A. Acellular collagen matrix as a possible "off the shelf" biomaterial for urethral repair. Urology. 1999;54:407–410. doi: 10.1016/s0090-4295(99)00179-x. [ DOI ] [ PubMed ] [ Google Scholar ]
- 8. Kim BS, Mooney DJ. Development of biocompatible synthetic extracellular matrices for tissue engineering. Trends Biotechnol. 1998;16:224–230. doi: 10.1016/s0167-7799(98)01191-3. [ DOI ] [ PubMed ] [ Google Scholar ]
- 9. Bergsma JE, Rozema FR, Bos RR, Boering G, de Bruijn WC, Pennings AJ. In vivo degradation and biocompatibility study of in vitro pre-degraded as-polymerized polyactide particles. Biomaterials. 1995;16:267–274. doi: 10.1016/0142-9612(95)93253-a. [ DOI ] [ PubMed ] [ Google Scholar ]
- 10. Li ST. Biologic biomaterials: tissue-derived biomateri-als (collagen) In: Brozino JD, editor. The Biomedical Engineering Handbook. Boca Raton, FL: CRS Press; 1995. pp. 627–647. [ Google Scholar ]
- 11. Silver FH, Pins G. Cell growth on collagen: a review of tissue engineering using scaffolds containing extracellular matrix. J Long Term Eff Med Implants. 1992;2:67–80. [ PubMed ] [ Google Scholar ]
- 12. Sams AE, Nixon AJ. Chondrocyte-laden collagen scaffolds for resurfacing extensive articular cartilage defects. Osteoarthritis Cartilage. 1995;3:47–59. doi: 10.1016/s1063-4584(05)80037-8. [ DOI ] [ PubMed ] [ Google Scholar ]
- 13. Smidsrød O, Skjåk-Braek G. Alginate as immobilization matrix for cells. Trends Biotechnol. 1990;8:71–78. doi: 10.1016/0167-7799(90)90139-o. [ DOI ] [ PubMed ] [ Google Scholar ]
- 14. Lim F, Sun AM. Microencapsulated islets as bioartificial endocrine pancreas. Science. 1980;210:908–910. doi: 10.1126/science.6776628. [ DOI ] [ PubMed ] [ Google Scholar ]
- 15. Gilding DK. Biodegradable polymers. In: Williams DF, editor. Biocompatibility of Clinical Implant Materials. Boca Raton, FL: CRC Press; 1981. pp. 209–232. [ Google Scholar ]
- 16. Mikos AG, Lyman MD, Freed LE, Langer R. Wetting of poly (L-lactic acid) and poly (DL-lactic-co-glycolic acid) foams for tissue culture. Biomaterials. 1994;15:55–58. doi: 10.1016/0142-9612(94)90197-x. [ DOI ] [ PubMed ] [ Google Scholar ]
- 17. Choi JS, Lee SJ, Christ GJ, Atala A, Yoo JJ. The influence of electrospun aligned poly (epsilon-caprolactone)/collagen nanofiber meshes on the formation of self-aligned skeletal muscle myotubes. Biomaterials. 2008;29:2899–2906. doi: 10.1016/j.biomaterials.2008.03.031. [ DOI ] [ PubMed ] [ Google Scholar ]
- 18. Lee SJ, Liu J, Oh SH, Soker S, Atala A, Yoo JJ. Development of a composite vascular scaffolding system that withstands physiological vascular conditions. Biomaterials. 2008;29:2891–2898. doi: 10.1016/j.biomaterials.2008.03.032. [ DOI ] [ PubMed ] [ Google Scholar ]
- 19. Pariente JL, Kim BS, Atala A. In vitro biocompatibility assessment of naturally derived and synthetic biomaterials using normal human urothelial cells. J Biomed Mater Res. 2001;55:33–39. doi: 10.1002/1097-4636(200104)55:1<33::aid-jbm50>3.0.co;2-7. [ DOI ] [ PubMed ] [ Google Scholar ]
- 20. Pariente JL, Kim BS, Atala A. In vitro biocompatibility evaluation of naturally derived and synthetic biomaterials using normal human bladder smooth muscle cells. J Urol. 2002;167:1867–1871. [ PubMed ] [ Google Scholar ]
- 21. Amiel GE, Atala A. Current and future modalities for functional renal replacement. Urol Clin North Am. 1999;26:235–246. doi: 10.1016/s0094-0143(99)80019-6. [ DOI ] [ PubMed ] [ Google Scholar ]
- 22. Amiel GE, Komura M, Shapira O, Yoo JJ, Yazdani S, Berry J, et al. Engineering of blood vessels from acellular collagen matrices coated with human endothelial cells. Tissue Eng. 2006;12:2355–2365. doi: 10.1089/ten.2006.12.2355. [ DOI ] [ PubMed ] [ Google Scholar ]
- 23. Yoo JJ, Park HJ, Lee I, Atala A. Autologous engineered cartilage rods for penile reconstruction. J Urol. 1999;162:1119–1121. doi: 10.1016/S0022-5347(01)68090-X. [ DOI ] [ PubMed ] [ Google Scholar ]
- 24. Atala A. Autologous cell transplantation for urologic reconstruction. J Urol. 1998;159:2–3. doi: 10.1016/s0022-5347(01)63994-6. [ DOI ] [ PubMed ] [ Google Scholar ]
- 25. Atala A. Bladder regeneration by tissue engineering. BJU Int. 2001;88:765–770. doi: 10.1046/j.1464-4096.2001.atala.2467.x. [ DOI ] [ PubMed ] [ Google Scholar ]
- 26. Atala A. Creation of bladder tissue in vitro and in vivo. A system for organ replacement. Adv Exp Med Biol. 1999;462:31–42. doi: 10.1007/978-1-4615-4737-2_3. [ DOI ] [ PubMed ] [ Google Scholar ]
- 27. Cilento BG, Freeman MR, Schneck FX, Retik AB, Atala A. Phenotypic and cytogenetic characterization of human bladder urothelia expanded in vitro. J Urol. 1994;152:665–670. doi: 10.1016/s0022-5347(17)32676-9. [ DOI ] [ PubMed ] [ Google Scholar ]
- 28. Oberpenning F, Meng J, Yoo JJ, Atala A. De novo reconstitution of a functional mammalian urinary bladder by tissue engineering. Nat Biotechnol. 1999;17:149–155. doi: 10.1038/6146. [ DOI ] [ PubMed ] [ Google Scholar ]
- 29. Scriven SD, Booth C, Thomas DF, Trejdosiewicz LK, Southgate J. Reconstitution of human urothelium from monolayer cultures. J Urol. 1997;158:1147–1152. doi: 10.1097/00005392-199709000-00115. [ DOI ] [ PubMed ] [ Google Scholar ]
- 30. Liebert M, Hubbel A, Chung M, Wedemeyer G, Lomax MI, Hegeman A, et al. Expression of mal is associated with urothelial differentiation in vitro: identification by differential display reverse-transcriptase polymerase chain reaction. Differentiation. 1997;61:177–185. doi: 10.1046/j.1432-0436.1997.6130177.x. [ DOI ] [ PubMed ] [ Google Scholar ]
- 31. Liebert M, Wedemeyer G, Abruzzo LV, Kunkel SL, Hammerberg C, Cooper KD, et al. Stimulated urothelial cells produce cytokines and express an activated cell surface antigenic phenotype. Semin Urol. 1991;9:124–130. [ PubMed ] [ Google Scholar ]
- 32. Puthenveettil JA, Burger MS, Reznikoff CA. Replicative senescence in human uroepithelial cells. Adv Exp Med Biol. 1999;462:83–91. doi: 10.1007/978-1-4615-4737-2_7. [ DOI ] [ PubMed ] [ Google Scholar ]
- 33. Freeman MR, Yoo JJ, Raab G, Soker S, Adam RM, Schneck FX, et al. Heparin-binding EGF-like growth factor is an autocrine growth factor for human urothelial cells and is synthesized by epithelial and smooth muscle cells in the human bladder. J Clin Invest. 1997;99:1028–1036. doi: 10.1172/JCI119230. [ DOI ] [ PMC free article ] [ PubMed ] [ Google Scholar ]
- 34. Nguyen HT, Park JM, Peters CA, Adam RM, Orsola A, Atala A, et al. Cell-specific activation of the HB-EGF and ErbB1 genes by stretch in primary human bladder cells. In Vitro Cell Dev Biol Anim. 1999;35:371–375. doi: 10.1007/s11626-999-0110-9. [ DOI ] [ PubMed ] [ Google Scholar ]
- 35. Harriss DR. Smooth muscle cell culture: a new approach to the study of human detrusor physiology and pathophysiology. Br J Urol. 1995;75(Suppl 1):18–26. [ PubMed ] [ Google Scholar ]
- 36. Brivanlou AH, Gage FH, Jaenisch R, Jessell T, Melton D, Rossant J. Stem cells. Setting standards for human embryonic stem cells. Science. 2003;300:913–916. doi: 10.1126/science.1082940. [ DOI ] [ PubMed ] [ Google Scholar ]
- 37. Richards M, Fong CY, Chan WK, Wong PC, Bongso A. Human feeders support prolonged undifferentiated growth of human inner cell masses and embryonic stem cells. Nat Biotechnol. 2002;20:933–936. doi: 10.1038/nbt726. [ DOI ] [ PubMed ] [ Google Scholar ]
- 38. Amit M, Shariki C, Margulets V, Itskovitz-Eldor J. Feeder layer- and serum-free culture of human embryonic stem cells. Biol Reprod. 2004;70:837–845. doi: 10.1095/biolreprod.103.021147. [ DOI ] [ PubMed ] [ Google Scholar ]
- 39. Reubinoff BE, Pera MF, Fong CY, Trounson A, Bongso A. Embryonic stem cell lines from human blastocysts: somatic differentiation in vitro. Nat Biotechnol. 2000;18:399–404. doi: 10.1038/74447. [ DOI ] [ PubMed ] [ Google Scholar ]
- 40. Reubinoff BE, Itsykson P, Turetsky T, Pera MF, Reinhartz E, Itzik A, et al. Neural progenitors from human embryonic stem cells. Nat Biotechnol. 2001;19:1134–1140. doi: 10.1038/nbt1201-1134. [ DOI ] [ PubMed ] [ Google Scholar ]
- 41. Schuldiner M, Eiges R, Eden A, Yanuka O, Itskovitz-Eldor J, Goldstein RS, et al. Induced neuronal differentiation of human embryonic stem cells. Brain Res. 2001;913:201–205. doi: 10.1016/s0006-8993(01)02776-7. [ DOI ] [ PubMed ] [ Google Scholar ]
- 42. Schuldiner M, Yanuka O, Itskovitz-Eldor J, Melton DA, Benvenisty N. Effects of eight growth factors on the differentiation of cells derived from human embryonic stem cells. Proc Natl Acad Sci U S A. 2000;97:11307–11312. doi: 10.1073/pnas.97.21.11307. [ DOI ] [ PMC free article ] [ PubMed ] [ Google Scholar ]
- 43. Zhang SC, Wernig M, Duncan ID, Brüstle O, Thomson JA. In vitro differentiation of transplantable neural precursors from human embryonic stem cells. Nat Biotechnol. 2001;19:1129–1133. doi: 10.1038/nbt1201-1129. [ DOI ] [ PubMed ] [ Google Scholar ]
- 44. Kaufman DS, Hanson ET, Lewis RL, Auerbach R, Thomson JA. Hematopoietic colony-forming cells derived from human embryonic stem cells. Proc Natl Acad Sci U S A. 2001;98:10716–10721. doi: 10.1073/pnas.191362598. [ DOI ] [ PMC free article ] [ PubMed ] [ Google Scholar ]
- 45. Kehat I, Kenyagin-Karsenti D, Snir M, Segev H, Amit M, Gepstein A, et al. Human embryonic stem cells can differentiate into myocytes with structural and functional properties of cardiomyocytes. J Clin Invest. 2001;108:407–414. doi: 10.1172/JCI12131. [ DOI ] [ PMC free article ] [ PubMed ] [ Google Scholar ]
- 46. Levenberg S, Golub JS, Amit M, Itskovitz-Eldor J, Langer R. Endothelial cells derived from human embryonic stem cells. Proc Natl Acad Sci U S A. 2002;99:4391–4396. doi: 10.1073/pnas.032074999. [ DOI ] [ PMC free article ] [ PubMed ] [ Google Scholar ]
- 47. Assady S, Maor G, Amit M, Itskovitz-Eldor J, Skorecki KL, Tzukerman M. Insulin production by human embryonic stem cells. Diabetes. 2001;50:1691–1697. doi: 10.2337/diabetes.50.8.1691. [ DOI ] [ PubMed ] [ Google Scholar ]
- 48. Itskovitz-Eldor J, Schuldiner M, Karsenti D, Eden A, Yanuka O, Amit M, et al. Differentiation of human embryonic stem cells into embryoid bodies compromising the three embryonic germ layers. Mol Med. 2000;6:88–95. [ PMC free article ] [ PubMed ] [ Google Scholar ]
- 49. Gurdon JB, Elsdale TR, Fischberg M. Sexually mature individuals of Xenopus laevis from the transplantation of single somatic nuclei. Nature. 1958;182:64–65. doi: 10.1038/182064a0. [ DOI ] [ PubMed ] [ Google Scholar ]
- 50. Campbell KH, McWhir J, Ritchie WA, Wilmut I. Sheep cloned by nuclear transfer from a cultured cell line. Nature. 1996;380:64–66. doi: 10.1038/380064a0. [ DOI ] [ PubMed ] [ Google Scholar ]
- 51. Wilmut I, Schnieke AE, McWhir J, Kind AJ, Campbell KH. Viable offspring derived from fetal and adult mammalian cells. Nature. 1997;385:810–813. doi: 10.1038/385810a0. [ DOI ] [ PubMed ] [ Google Scholar ]
- 52. Cibelli JB, Stice SL, Golueke PJ, Kane JJ, Jerry J, Blackwell C, et al. Cloned transgenic calves produced from nonquiescent fetal fibroblasts. Science. 1998;280:1256–1258. doi: 10.1126/science.280.5367.1256. [ DOI ] [ PubMed ] [ Google Scholar ]
- 53. Baguisi A, Behboodi E, Melican DT, Pollock JS, Destrempes MM, Cammuso C, et al. Production of goats by somatic cell nuclear transfer. Nat Biotechnol. 1999;17:456–461. doi: 10.1038/8632. [ DOI ] [ PubMed ] [ Google Scholar ]
- 54. Wakayama T, Perry AC, Zuccotti M, Johnson KR, Yanagimachi R. Full-term development of mice from enucleated oocytes injected with cumulus cell nuclei. Nature. 1998;394:369–374. doi: 10.1038/28615. [ DOI ] [ PubMed ] [ Google Scholar ]
- 55. Betthauser J, Forsberg E, Augenstein M, Childs L, Eilertsen K, Enos J, et al. Production of cloned pigs from in vitro systems. Nat Biotechnol. 2000;18:1055–1059. doi: 10.1038/80242. [ DOI ] [ PubMed ] [ Google Scholar ]
- 56. De Sousa PA, Dobrinsky JR, Zhu J, Archibald AL, Ainslie A, Bosma W, et al. Somatic cell nuclear transfer in the pig: control of pronuclear formation and integration with improved methods for activation and maintenance of pregnancy. Biol Reprod. 2002;66:642–650. doi: 10.1095/biolreprod66.3.642. [ DOI ] [ PubMed ] [ Google Scholar ]
- 57. Colman A, Kind A. Therapeutic cloning: concepts and practicalities. Trends Biotechnol. 2000;18:192–196. doi: 10.1016/s0167-7799(00)01434-7. [ DOI ] [ PubMed ] [ Google Scholar ]
- 58. Vogelstein B, Alberts B, Shine K. Genetics. Please don't call it cloning! Science. 2002;295:1237. doi: 10.1126/science.1070247. [ DOI ] [ PubMed ] [ Google Scholar ]
- 59. Hochedlinger K, Rideout WM, Kyba M, Daley GQ, Blelloch R, Jaenisch R. Nuclear transplantation, embryonic stem cells and the potential for cell therapy. Hematol J. 2004;5(Suppl 3):S114–S117. doi: 10.1038/sj.thj.6200435. [ DOI ] [ PubMed ] [ Google Scholar ]
- 60. Lanza RP, Cibelli JB, West MD. Prospects for the use of nuclear transfer in human transplantation. Nat Biotechnol. 1999;17:1171–1174. doi: 10.1038/70709. [ DOI ] [ PubMed ] [ Google Scholar ]
- 61. Lanza RP, Cibelli JB, West MD, Dorff E, Tauer C, Green RM. The ethical reasons for stem cell research. Science. 2001;292:1299. doi: 10.1126/science.292.5520.1299b. [ DOI ] [ PubMed ] [ Google Scholar ]
- 62. Rideout WM, 3rd, Eggan K, Jaenisch R. Nuclear cloning and epigenetic reprogramming of the genome. Science. 2001;293:1093–1098. doi: 10.1126/science.1063206. [ DOI ] [ PubMed ] [ Google Scholar ]
- 63. Solter D. Mammalian cloning: advances and limitations. Nat Rev Genet. 2000;1:199–207. doi: 10.1038/35042066. [ DOI ] [ PubMed ] [ Google Scholar ]
- 64. Hochedlinger K, Jaenisch R. Nuclear transplantation: lessons from frogs and mice. Curr Opin Cell Biol. 2002;14:741–748. doi: 10.1016/s0955-0674(02)00380-0. [ DOI ] [ PubMed ] [ Google Scholar ]
- 65. Dinnyés A, De Sousa P, King T, Wilmut I. Somatic cell nuclear transfer: recent progress and challenges. Cloning Stem Cells. 2002;4:81–90. doi: 10.1089/153623002753632075. [ DOI ] [ PubMed ] [ Google Scholar ]
- 66. Takahashi K, Yamanaka S. Induction of pluripotent stem cells from mouse embryonic and adult fibroblast cultures by defined factors. Cell. 2006;126:663–676. doi: 10.1016/j.cell.2006.07.024. [ DOI ] [ PubMed ] [ Google Scholar ]
- 67. Wernig M, Meissner A, Foreman R, Brambrink T, Ku M, Hochedlinger K, et al. In vitro reprogramming of fibroblasts into a pluripotent ES-cell-like state. Nature. 2007;448:318–324. doi: 10.1038/nature05944. [ DOI ] [ PubMed ] [ Google Scholar ]
- 68. Takahashi K, Tanabe K, Ohnuki M, Narita M, Ichisaka T, Tomoda K, et al. Induction of pluripotent stem cells from adult human fibroblasts by defined factors. Cell. 2007;131:861–872. doi: 10.1016/j.cell.2007.11.019. [ DOI ] [ PubMed ] [ Google Scholar ]
- 69. Yu J, Vodyanik MA, Smuga-Otto K, Antosiewicz-Bourget J, Frane JL, Tian S, et al. Induced pluripotent stem cell lines derived from human somatic cells. Science. 2007;318:1917–1920. doi: 10.1126/science.1151526. [ DOI ] [ PubMed ] [ Google Scholar ]
- 70. De Coppi P, Bartsch G, Jr, Siddiqui MM, Xu T, Santos CC, Perin L, et al. Isolation of amniotic stem cell lines with potential for therapy. Nat Biotechnol. 2007;25:100–106. doi: 10.1038/nbt1274. [ DOI ] [ PubMed ] [ Google Scholar ]
- 71. Ballas CB, Zielske SP, Gerson SL. Adult bone marrow stem cells for cell and gene therapies: implications for greater use. J Cell Biochem Suppl. 2002;38:20–28. doi: 10.1002/jcb.10127. [ DOI ] [ PubMed ] [ Google Scholar ]
- 72. McCulloch EA, Till JE. Proliferation of hemopoietic colony-forming cells transplanted into irradiated mice. Radiat Res. 1964;22:383–397. [ PubMed ] [ Google Scholar ]
- 73. Jiao J, Chen DF. Induction of neurogenesis in nonconventional neurogenic regions of the adult central nervous system by niche astrocyte-produced signals. Stem Cells. 2008;26:1221–1230. doi: 10.1634/stemcells.2007-0513. [ DOI ] [ PMC free article ] [ PubMed ] [ Google Scholar ]
- 74. Taupin P. Therapeutic potential of adult neural stem cells. Recent Pat CNS Drug Discov. 2006;1:299–303. doi: 10.2174/157488906778773670. [ DOI ] [ PubMed ] [ Google Scholar ]
- 75. Jensen UB, Yan X, Triel C, Woo SH, Christensen R, Owens DM. A distinct population of clonogenic and multipotent murine follicular keratinocytes residing in the upper isthmus. J Cell Sci. 2008;121:609–617. doi: 10.1242/jcs.025502. [ DOI ] [ PMC free article ] [ PubMed ] [ Google Scholar ]
- 76. Crisan M, Casteilla L, Lehr L, Carmona M, Paoloni-Giacobino A, Yap S, et al. A reservoir of brown adipocyte progenitors in human skeletal muscle. Stem Cells. 2008;26:2425–2433. doi: 10.1634/stemcells.2008-0325. [ DOI ] [ PubMed ] [ Google Scholar ]
- 77. Weiner LP. Definitions and criteria for stem cells. Methods Mol Biol. 2008;438:3–8. doi: 10.1007/978-1-59745-133-8_1. [ DOI ] [ PubMed ] [ Google Scholar ]
- 78. Devine SM. Mesenchymal stem cells: will they have a role in the clinic? J Cell Biochem Suppl. 2002;38:73–79. doi: 10.1002/jcb.10046. [ DOI ] [ PubMed ] [ Google Scholar ]
- 79. Jiang Y, Jahagirdar BN, Reinhardt RL, Schwartz RE, Keene CD, Ortiz-Gonzalez XR, et al. Pluripotency of mesenchymal stem cells derived from adult marrow. Nature. 2002;418:41–49. doi: 10.1038/nature00870. [ DOI ] [ PubMed ] [ Google Scholar ]
- 80. Caplan AI. Adult mesenchymal stem cells for tissue engineering versus regenerative medicine. J Cell Physiol. 2007;213:341–347. doi: 10.1002/jcp.21200. [ DOI ] [ PubMed ] [ Google Scholar ]
- 81. da Silva Meirelles L, Caplan AI, Nardi NB. In search of the in vivo identity of mesenchymal stem cells. Stem Cells. 2008;26:2287–2299. doi: 10.1634/stemcells.2007-1122. [ DOI ] [ PubMed ] [ Google Scholar ]
- 82. Duan X, Chang JH, Ge S, Faulkner RL, Kim JY, Kitabatake Y, et al. Disrupted-In-Schizophrenia 1 regulates integration of newly generated neurons in the adult brain. Cell. 2007;130:1146–1158. doi: 10.1016/j.cell.2007.07.010. [ DOI ] [ PMC free article ] [ PubMed ] [ Google Scholar ]
- 83. Luttun A, Ross JJ, Verfaillie C, Aranguren X, Prosper F. Current Protocols in Immunology. Hoboken, NJ: John Wiley and Sons, Inc.; 2006. Unit 22F.9: Differentiation of multipotent adult progenitor cells into functional endothelial and smooth muscle cells. [ DOI ] [ PubMed ] [ Google Scholar ]
- 84. Mimeault M, Batra SK. Recent progress on tissue-resident adult stem cell biology and their therapeutic implications. Stem Cell Rev. 2008;4:27–49. doi: 10.1007/s12015-008-9008-2. [ DOI ] [ PMC free article ] [ PubMed ] [ Google Scholar ]
- 85. Ikeda E, Yagi K, Kojima M, Yagyuu T, Ohshima A, Sobajima S, et al. Multipotent cells from the human third molar: feasibility of cell-based therapy for liver disease. Differentiation. 2008;76:495–505. doi: 10.1111/j.1432-0436.2007.00245.x. [ DOI ] [ PubMed ] [ Google Scholar ]
- 86. Nolen-Walston RD, Kim CF, Mazan MR, Ingenito EP, Gruntman AM, Tsai L, et al. Cellular kinetics and modeling of bronchioalveolar stem cell response during lung regeneration. Am J Physiol Lung Cell Mol Physiol. 2008;294:L1158–L1165. doi: 10.1152/ajplung.00298.2007. [ DOI ] [ PMC free article ] [ PubMed ] [ Google Scholar ]
- 87. in 't Anker PS, Noort WA, Scherjon SA, Kleijburg-van der Keur C, Kruisselbrink AB, van Bezooijen RL, et al. Mesenchymal stem cells in human second-trimester bone marrow, liver, lung, and spleen exhibit a similar immunophenotype but a heterogeneous multilineage differentiation potential. Haematologica. 2003;88:845–852. [ PubMed ] [ Google Scholar ]
- 88. Wilson A, Butler PE, Seifalian AM. Adipose-derived stem cells for clinical applications: a review. Cell Prolif. 2011;44:86–98. doi: 10.1111/j.1365-2184.2010.00736.x. [ DOI ] [ PMC free article ] [ PubMed ] [ Google Scholar ]
- 89. Mizuno H. Adipose-derived stem and stromal cells for cell-based therapy: current status of preclinical studies and clinical trials. Curr Opin Mol Ther. 2010;12:442–449. [ PubMed ] [ Google Scholar ]
- 90. Hristov M, Zernecke A, Schober A, Weber C. Adult progenitor cells in vascular remodeling during atherosclerosis. Biol Chem. 2008;389:837–844. doi: 10.1515/BC.2008.093. [ DOI ] [ PubMed ] [ Google Scholar ]
- 91. Zhou Q, Brown J, Kanarek A, Rajagopal J, Melton DA. In vivo reprogramming of adult pancreatic exocrine cells to beta-cells. Nature. 2008;455:627–632. doi: 10.1038/nature07314. [ DOI ] [ PMC free article ] [ PubMed ] [ Google Scholar ]
- 92. Torrente Y, Tremblay JP, Pisati F, Belicchi M, Rossi B, Sironi M, et al. Intraarterial injection of muscle-derived CD34(+)Sca-1(+) stem cells restores dystrophin in mdx mice. J Cell Biol. 2001;152:335–348. doi: 10.1083/jcb.152.2.335. [ DOI ] [ PMC free article ] [ PubMed ] [ Google Scholar ]
- 93. Atala A, Cima LG, Kim W, Paige KT, Vacanti JP, Retik AB, et al. Injectable alginate seeded with chondrocytes as a potential treatment for vesicoureteral reflux. J Urol. 1993;150:745–747. doi: 10.1016/s0022-5347(17)35603-3. [ DOI ] [ PubMed ] [ Google Scholar ]
- 94. Atala A, Kim W, Paige KT, Vacanti CA, Retik AB. Endoscopic treatment of vesicoureteral reflux with a chondrocyte-alginate suspension. J Urol. 1994;152:641–643. doi: 10.1016/s0022-5347(17)32671-x. [ DOI ] [ PubMed ] [ Google Scholar ]
- 95. Diamond DA, Caldamone AA. Endoscopic correction of vesicoureteral reflux in children using autologous chondrocytes: preliminary results. J Urol. 1999;162:1185–1188. doi: 10.1016/S0022-5347(01)68124-2. [ DOI ] [ PubMed ] [ Google Scholar ]
- 96. Bent AE, Tutrone RT, McLennan MT, Lloyd LK, Kennelly MJ, Badlani G. Treatment of intrinsic sphincter deficiency using autologous ear chondrocytes as a bulking agent. Neurourol Urodyn. 2001;20:157–165. doi: 10.1002/1520-6777(2001)20:2<157::aid-nau18>3.0.co;2-a. [ DOI ] [ PubMed ] [ Google Scholar ]
- 97. Chen F, Yoo JJ, Atala A. Experimental and clinical experience using tissue regeneration for urethral reconstruction. World J Urol. 2000;18:67–70. doi: 10.1007/s003450050012. [ DOI ] [ PubMed ] [ Google Scholar ]
- 98. Kropp BP, Ludlow JK, Spicer D, Rippy MK, Badylak SF, Adams MC, et al. Rabbit urethral regeneration using small intestinal submucosa onlay grafts. Urology. 1998;52:138–142. doi: 10.1016/s0090-4295(98)00114-9. [ DOI ] [ PubMed ] [ Google Scholar ]
- 99. Sievert KD, Bakircioglu ME, Nunes L, Tu R, Dahiya R, Tanagho EA. Homologous acellular matrix graft for urethral reconstruction in the rabbit: histological and functional evaluation. J Urol. 2000;163:1958–1965. [ PubMed ] [ Google Scholar ]
- 100. El-Kassaby AW, Retik AB, Yoo JJ, Atala A. Urethral stricture repair with an off-the-shelf collagen matrix. J Urol. 2003;169:170–173. doi: 10.1016/S0022-5347(05)64060-8. [ DOI ] [ PubMed ] [ Google Scholar ]
- 101. De Filippo RE, Yoo JJ, Atala A. Urethral replacement using cell seeded tubularized collagen matrices. J Urol. 2002;168:1789–1792. doi: 10.1097/01.ju.0000027662.69103.72. [ DOI ] [ PubMed ] [ Google Scholar ]
- 102. Dorin RP, Pohl HG, De Filippo RE, Yoo JJ, Atala A. Tubularized urethral replacement with unseeded matrices: what is the maximum distance for normal tissue regeneration? World J Urol. 2008;26:323–326. doi: 10.1007/s00345-008-0316-6. [ DOI ] [ PubMed ] [ Google Scholar ]
- 103. Raya-Rivera A, Esquiliano DR, Yoo JJ, Lopez-Bayghen E, Soker S, Atala A. Tissue-engineered autologous urethras for patients who need reconstruction: an observational study. Lancet. 2011;377:1175–1182. doi: 10.1016/S0140-6736(10)62354-9. [ DOI ] [ PMC free article ] [ PubMed ] [ Google Scholar ]
- 104. Atala A, Freeman MR, Vacanti JP, Shepard J, Retik AB. Implantation in vivo and retrieval of artificial structures consisting of rabbit and human urothelium and human bladder muscle. J Urol. 1993;150:608–612. doi: 10.1016/s0022-5347(17)35561-1. [ DOI ] [ PubMed ] [ Google Scholar ]
- 105. Jayo MJ, Jain D, Ludlow JW, Payne R, Wagner BJ, McLorie G, et al. Long-term durability, tissue regeneration and neo-organ growth during skeletal maturation with a neo-bladder augmentation construct. Regen Med. 2008;3:671–682. doi: 10.2217/17460751.3.5.671. [ DOI ] [ PubMed ] [ Google Scholar ]
- 106. Jayo MJ, Jain D, Wagner BJ, Bertram TA. Early cellular and stromal responses in regeneration versus repair of a mammalian bladder using autologous cell and biodegradable scaffold technologies. J Urol. 2008;180:392–397. doi: 10.1016/j.juro.2008.02.039. [ DOI ] [ PubMed ] [ Google Scholar ]
- 107. Atala A, Bauer SB, Soker S, Yoo JJ, Retik AB. Tissue-engineered autologous bladders for patients needing cystoplasty. Lancet. 2006;367:1241–1246. doi: 10.1016/S0140-6736(06)68438-9. [ DOI ] [ PubMed ] [ Google Scholar ]
- 108. Matsumura G, Miyagawa-Tomita S, Shin'oka T, Ikada Y, Kurosawa H. First evidence that bone marrow cells contribute to the construction of tissue-engineered vascular autografts in vivo. Circulation. 2003;108:1729–1734. doi: 10.1161/01.CIR.0000092165.32213.61. [ DOI ] [ PubMed ] [ Google Scholar ]
- 109. Watanabe M, Shin'oka T, Tohyama S, Hibino N, Konuma T, Matsumura G, et al. Tissue-engineered vascular autograft: inferior vena cava replacement in a dog model. Tissue Eng. 2001;7:429–439. doi: 10.1089/10763270152436481. [ DOI ] [ PubMed ] [ Google Scholar ]
- 110. Shinoka T, Breuer CK, Tanel RE, Zund G, Miura T, Ma PX, et al. Tissue engineering heart valves: valve leaflet replacement study in a lamb model. Ann Thorac Surg. 1995;60(6 Suppl):S513–S516. doi: 10.1016/0003-4975(95)00733-4. [ DOI ] [ PubMed ] [ Google Scholar ]
- 111. Shinoka T, Shum-Tim D, Ma PX, Tanel RE, Isogai N, Langer R, et al. Creation of viable pulmonary artery autografts through tissue engineering. J Thorac Cardiovasc Surg. 1998;115:536–545. doi: 10.1016/S0022-5223(98)70315-0. [ DOI ] [ PubMed ] [ Google Scholar ]
- 112. Shinoka T, Shum-Tim D, Ma PX, Tanel RE, Langer R, Vacanti JP, et al. Tissue-engineered heart valve leaflets: does cell origin affect outcome? Circulation. 1997;96(9 Suppl):II-102–II-107. [ PubMed ] [ Google Scholar ]
- 113. Shin'oka T, Imai Y, Ikada Y. Transplantation of a tissue-engineered pulmonary artery. N Engl J Med. 2001;344:532–533. doi: 10.1056/NEJM200102153440717. [ DOI ] [ PubMed ] [ Google Scholar ]
- 114. L'Heureux N, McAllister TN, de la Fuente LM. Tissue-engineered blood vessel for adult arterial revascularization. N Engl J Med. 2007;357:1451–1453. doi: 10.1056/NEJMc071536. [ DOI ] [ PubMed ] [ Google Scholar ]
- 115. McAllister TN, Maruszewski M, Garrido SA, Wystrychowski W, Dusserre N, Marini A, et al. Effectiveness of haemodialysis access with an autologous tissue-engineered vascular graft: a multicentre cohort study. Lancet. 2009;373:1440–1446. doi: 10.1016/S0140-6736(09)60248-8. [ DOI ] [ PubMed ] [ Google Scholar ]
- 116. Fuchs JR, Terada S, Ochoa ER, Vacanti JP, Fauza DO. Fetal tissue engineering: in utero tracheal augmentation in an ovine model. J Pediatr Surg. 2002;37:1000–1006. doi: 10.1053/jpsu.2002.33829. [ DOI ] [ PubMed ] [ Google Scholar ]
- 117. Macchiarini P, Jungebluth P, Go T, Asnaghi MA, Rees LE, Cogan TA, et al. Clinical transplantation of a tissue-engineered airway. Lancet. 2008;372:2023–2030. doi: 10.1016/S0140-6736(08)61598-6. [ DOI ] [ PubMed ] [ Google Scholar ]
- 118. Auchincloss H, Bonventre JV. Transplanting cloned cells into therapeutic promise. Nat Biotechnol. 2002;20:665–666. doi: 10.1038/nbt0702-665. [ DOI ] [ PubMed ] [ Google Scholar ]
- 119. Lanza RP, Chung HY, Yoo JJ, Wettstein PJ, Blackwell C, Borson N, et al. Generation of histocompatible tissues using nuclear transplantation. Nat Biotechnol. 2002;20:689–696. doi: 10.1038/nbt703. [ DOI ] [ PubMed ] [ Google Scholar ]
- 120. Nakase Y, Nakamura T, Kin S, Nakashima S, Yoshikawa T, Kuriu Y, et al. Endocrine cell and nerve regeneration in autologous in situ tissue-engineered small intestine. J Surg Res. 2007;137:61–68. doi: 10.1016/j.jss.2006.06.019. [ DOI ] [ PubMed ] [ Google Scholar ]
- 121. Auger FA, Berthod F, Moulin V, Pouliot R, Germain L. Tissue-engineered skin substitutes: from in vitro constructs to in vivo applications. Biotechnol Appl Biochem. 2004;39:263–275. doi: 10.1042/BA20030229. [ DOI ] [ PubMed ] [ Google Scholar ]
- 122. Suuronen EJ, McLaughlin CR, Stys PK, Nakamura M, Munger R, Griffith M. Functional innervation in tissue engineered models for in vitro study and testing purposes. Toxicol Sci. 2004;82:525–533. doi: 10.1093/toxsci/kfh270. [ DOI ] [ PubMed ] [ Google Scholar ]
- 123. Burdick JA, Ward M, Liang E, Young MJ, Langer R. Stimulation of neurite outgrowth by neurotrophins delivered from degradable hydrogels. Biomaterials. 2006;27:452–459. doi: 10.1016/j.biomaterials.2005.06.034. [ DOI ] [ PubMed ] [ Google Scholar ]
- 124. Dennis RG, Dow DE. Excitability of skeletal muscle during development, denervation, and tissue culture. Tissue Eng. 2007;13:2395–2404. doi: 10.1089/ten.2006.0367. [ DOI ] [ PubMed ] [ Google Scholar ]
- 125. Popel AS. Theory of oxygen transport to tissue. Crit Rev Biomed Eng. 1989;17:257–321. [ PMC free article ] [ PubMed ] [ Google Scholar ]
- 126. Muschler GF, Nakamoto C, Griffith LG. Engineering principles of clinical cell-based tissue engineering. J Bone Joint Surg Am. 2004;86:1541–1558. doi: 10.2106/00004623-200407000-00029. [ DOI ] [ PubMed ] [ Google Scholar ]
- 127. Risau W. Mechanisms of angiogenesis. Nature. 1997;386:671–674. doi: 10.1038/386671a0. [ DOI ] [ PubMed ] [ Google Scholar ]
- 128. Khong YM, Zhang J, Zhou S, Cheung C, Doberstein K, Samper V, et al. Novel intra-tissue perfusion system for culturing thick liver tissue. Tissue Eng. 2007;13:2345–2356. doi: 10.1089/ten.2007.0040. [ DOI ] [ PubMed ] [ Google Scholar ]
- 129. Iyer RK, Radisic M, Cannizzaro C, Vunjak-Novakovic G. Synthetic oxygen carriers in cardiac tissue engineering. Artif Cells Blood Substit Immobil Biotechnol. 2007;35:135–148. doi: 10.1080/10731190600974988. [ DOI ] [ PubMed ] [ Google Scholar ]
- 130. Radisic M, Park H, Chen F, Salazar-Lazzaro JE, Wang Y, Dennis R, et al. Biomimetic approach to cardiac tissue engineering: oxygen carriers and channeled scaffolds. Tissue Eng. 2006;12:2077–2091. doi: 10.1089/ten.2006.12.2077. [ DOI ] [ PubMed ] [ Google Scholar ]
- 131. Harrison BS, Eberli D, Lee SJ, Atala A, Yoo JJ. Oxygen producing biomaterials for tissue regeneration. Biomaterials. 2007;28:4628–4634. doi: 10.1016/j.biomaterials.2007.07.003. [ DOI ] [ PubMed ] [ Google Scholar ]
- 132. Tsang VL, Bhatia SN. Fabrication of three-dimensional tissues. Adv Biochem Eng Biotechnol. 2007;103:189–205. doi: 10.1007/10_010. [ DOI ] [ PubMed ] [ Google Scholar ]
- 133. Ott HC, Matthiesen TS, Goh SK, Black LD, Kren SM, Netoff TI, et al. Perfusion-decellularized matrix: using nature's platform to engineer a bioartificial heart. Nat Med. 2008;14:213–221. doi: 10.1038/nm1684. [ DOI ] [ PubMed ] [ Google Scholar ]
- 134. Baptista PM, Siddiqui MM, Lozier G, Rodriguez SR, Atala A, Soker S. The use of whole organ decellularization for the generation of a vascularized liver organoid. Hepatology. 2010 doi: 10.1002/hep.24067. [ DOI ] [ PubMed ] [ Google Scholar ]
- 135. Nahmias Y, Schwartz RE, Verfaillie CM, Odde DJ. Laser-guided direct writing for three-dimensional tissue engineering. Biotechnol Bioeng. 2005;92:129–136. doi: 10.1002/bit.20585. [ DOI ] [ PubMed ] [ Google Scholar ]
- 136. Xu T, Jin J, Gregory C, Hickman JJ, Boland T. Inkjet printing of viable mammalian cells. Biomaterials. 2005;26:93–99. doi: 10.1016/j.biomaterials.2004.04.011. [ DOI ] [ PubMed ] [ Google Scholar ]
- 137. Xu T, Olson J, Zhao W, Atala A, Zhu JM, Yoo JJ. Characterization of cell constructs generated with inkjet printing technology using in vivo magnetic resonance imaging. J Manuf Sci Eng. 2008;130:021013. [ Google Scholar ]
- 138. Stankus JJ, Soletti L, Fujimoto K, Hong Y, Vorp DA, Wagner WR. Fabrication of cell microintegrated blood vessel constructs through electrohydrodynamic atomization. Biomaterials. 2007;28:2738–2746. doi: 10.1016/j.biomaterials.2007.02.012. [ DOI ] [ PMC free article ] [ PubMed ] [ Google Scholar ]
- 139. Landman KA, Cai AQ. Cell proliferation and oxygen diffusion in a vascularising scaffold. Bull Math Biol. 2007;69:2405–2428. doi: 10.1007/s11538-007-9225-x. [ DOI ] [ PubMed ] [ Google Scholar ]
- 140. Nomi M, Atala A, Coppi PD, Soker S. Principals of neovascularization for tissue engineering. Mol Aspects Med. 2002;23:463–483. doi: 10.1016/s0098-2997(02)00008-0. [ DOI ] [ PubMed ] [ Google Scholar ]
- 141. Sellaro TL, Ravindra AK, Stolz DB, Badylak SF. Maintenance of hepatic sinusoidal endothelial cell phenotype in vitro using organ-specific extracellular matrix scaffolds. Tissue Eng. 2007;13:2301–2310. doi: 10.1089/ten.2006.0437. [ DOI ] [ PubMed ] [ Google Scholar ]
- 142. Lutolf MP, Gilbert PM, Blau HM. Designing materials to direct stem-cell fate. Nature. 2009;462:433–441. doi: 10.1038/nature08602. [ DOI ] [ PMC free article ] [ PubMed ] [ Google Scholar ]
- View on publisher site
- PDF (365.3 KB)
- Collections

Similar articles
Cited by other articles, links to ncbi databases.
- Download .nbib .nbib
- Format: AMA APA MLA NLM
Add to Collections
- Open access
- Published: 19 December 2024
Convergence of tissue engineering and sustainable development goals
- Naresh Kasoju 1 &
- Anagha Sunilkumar 1
Biotechnology for Sustainable Materials volume 1 , Article number: 20 ( 2024 ) Cite this article
185 Accesses
Metrics details
The convergence of sustainability with medical innovation in tissue engineering represents a pivotal advancement in both biomedical science and environmental stewardship. This review article provides a comprehensive overview of how tissue engineering integrates sustainable practices while advancing medical treatments. It delves into the core components of tissue engineering, focusing on the development and application of biomaterials such as polymers, metals, ceramics, composites, and decellularized materials. The article also explores cutting-edge technologies like bioprinting, electrospinning, cell sheet engineering, and microfluidics, highlighting their contributions to creating effective and eco-friendly medical solutions. A significant portion of the review is dedicated to sustainability and the Sustainable Development Goals (SDGs), with a particular emphasis on SDG 3 (Good Health and Well-being) and its intersection with tissue engineering. The covers other relevant SDGs, demonstrating how sustainable biomaterials and technologies contribute to broader objectives such as SDG 4, 5, 8, 9, 10, 11, 12, 17. Additionally, the discussion extends to other indirectly relevant SDGs such as SDG 1, 2, 6, 7, 13, 14, 15, 16. The article concludes with a summary of current advancements and prospects, emphasizing the importance of integrating eco-conscious approaches in tissue engineering to achieve a sustainable and innovative medical future.
Graphical Abstract

Introduction
The Sustainable Development Goals (SDGs) act as a global framework for achieving a more inclusive, equitable, and sustainable future [ 1 ]. Tissue engineering domain which emerged in the late 1980s, to create living, functional tissues in the laboratory for implantation in patients, thereby advancing regenerative medicine and personalized healthcare solutions [ 2 ]. Initially conceptualized to match the mechanical properties of native tissues and minimize harmful host responses, tissue engineering has significantly evolved, focusing now on creating constructs that not only integrate seamlessly with the body but also support functional restoration through biological compatibility and sustainability. The advent of tissue engineering has coincided with a growing global emphasis on sustainability, aligning with the SDGs established by the United Nations. The integration of sustainable practices in tissue engineering involves the use of eco-friendly materials in scaffold fabrication, green manufacturing processes, and the development of biodegradable and biocompatible biomaterials. These efforts underscore the importance of innovative research, collaborative partnerships, and regulatory support in advancing both the field of tissue engineering and global sustainability goals.
The review starts with an overview of tissue engineering, which includes an introduction, key aspects, and applications of tissue engineering. Following this, the review describe an overview of biomaterials, trends in biomaterials and the critical role of biomaterials in tissue engineering. Then, the review outlines the sustainable technologies involved in tissue engineering, by explaining the two different approaches of tissue engineering, the critical role of scaffolds in tissue engineering, and technological trends in tissue engineering. Then, the review discusses sustainability and SDGs as per the United Nation’s 2030 Agenda for Sustainable Development. It further provides deeper insights into sustainability in different fields like health care, biomedical engineering, and tissue engineering. Subsequently, this review explores how different biomaterials and tissue engineering technologies address sustainability issues. The review also analyses the biomaterials and tissue engineering technologies that follow 17 sustainable development guidelines. Finally, the review ends a summary and future perspectives. This review attempts to integrate how various aspects of biomaterials and innovative technologies within the field of tissue engineering align with sustainable development goals, particularly SDG 3, and thus highlight the convergence of sustainability with medical innovation.
Tissue engineering
Introduction to tissue engineering.
Tissue engineering is an interdisciplinary field that combines the concepts of life sciences, engineering, and medicine to develop biological substitutes capable of repairing, maintaining, or improving tissue function (Fig. 1 ). This field aims to create living, functional tissues in the laboratory that can be implanted into patients to restore normal function or facilitate healing, thus offering significant potential for advancing regenerative medicine and personalized healthcare solutions [ 2 ]. Tissue engineering was first introduced in the late 1980s in a meeting held by the National Science Foundation in the USA [ 3 ], while the paper entitled “Functional Organ Replacement: The New Technology of Tissue Engineering” in the year 1991 was first to use the term tissue engineering as it is known presently [ 4 ]. Tissue engineering can be achieved through ex vivo or in situ strategies, each with its own set of challenges and opportunities. Ex vivo strategies involve engineering tissue constructs in the lab before implantation, while in situ strategies involve delivering cells and/or a bioscaffold directly to the defect site to stimulate tissue repair [ 5 ]. As an industry, tissue engineering has experienced significant growth and reasonable commercial success in recent years [ 6 ]. Classically, tissue engineering recapitulates tissue and organ formation in our body to varying degrees, bringing together cells in a three-dimensional (3D) fabricated environment where appropriate signals are provided for tissue formation. In parallel, the evolution of tissue engineering research is intertwined with progress in other fields through the examination of cell function and behavior in isolated biomimetic microenvironments [ 7 ]. tissue engineering applications now extend beyond regeneration strategies alone, operating as a platform for modifiable, physiologically representative in vitro models [ 8 ].

Cells, scaffolds and growth factors, popularly known as the triad, are key components of tissue engineering, and though a variety of approaches it is possible to engineer a number of tissue types including hard, soft, sensory and other tissues
Key aspects of tissue engineering
TE requires a triad of components: (1) Harvested and dissociated cells from the donor tissue; (2) scaffolds made of biomaterials on which cells are attached and cultured, then implanted at the desired site in functioning tissue; and (3) growth factors that promote and/or prevent cell adhesion, proliferation, migration, and differentiation by up-regulating or down-regulating the synthesis of protein, growth factors, and receptors. A relevant selection of cells, a biomaterial scaffold, and the presence of appropriate signals coordinate to ultimately recreate tissue to address the limitations of current treatments for tissue and organ damage, such as donor shortages and rejection issues. The interaction between the tissue engineering triad is necessary to optimize the development of tissue in vitro [ 2 ]. These scaffolds can be fabricated from various materials, including biodegradable polymers, which degrade over time to allow for tissue integration and remodeling. The architecture of scaffolds, including their porosity and mechanical properties, was tailored to match the requirements of specific tissues, influencing cell behavior and subsequent tissue formation [ 9 ]. Cells used in tissue engineering can be derived from different sources, including embryonic stem cells, bone marrow-derived mesenchymal stem cells (MSCs), and cord-derived MSCs. These cells are capable of differentiating into various cell types, making them suitable for regenerating a wide range of tissues. The choice of cell type depends on the target tissue and the specific requirements for regeneration. Signalling molecules, including growth factors and cytokines, play a pivotal role in regulating cellular activities such as proliferation, migration, and differentiation. These molecules can be incorporated into the scaffolds to provide localized and sustained delivery, enhancing the regenerative potential of the engineered tissue. The controlled release of these signalling molecules from the scaffold ensures that cells receive the necessary cues for appropriate tissue development. Together these three components form the foundation of tissue engineering strategies aimed at restoring, maintaining, or improving tissue function [ 10 ].
Applications of tissue engineering
Tissue engineering has catered to create constructs that can effectively replace or support the functions of native tissues. The applications help in the regeneration of skin, bone, cardiac, liver, and nerve tissues. Skin tissue engineering was one of the most advanced areas, focusing on creating grafts for burn victims and patients with chronic wounds. Engineered skin constructs like Apligraf® and Dermagraft® are already in clinical use [ 11 ]. Additionally, advancements in 3D bioprinting allow for the precise deposition of cells and biomaterials to create layered skin structures that mimic the complexity of natural skin [ 12 ]. Bone tissue engineering aims to address issues such as fractures, osteoporosis, and bone defects. Techniques involve the use of biocompatible scaffolds made from materials like hydroxyapatite, tricalcium phosphate, and bioactive glasses, which provide structural support and promote osteogenesis [ 13 ]. Stem cells, particularly MSCs, are commonly used to differentiate into osteoblasts. The scaffolds are often enhanced with growth factors like bone morphogenic protein-2 to stimulate bone growth [ 14 ]. Cardiac tissue engineering addresses heart diseases and myocardial infarction by developing constructs that can repair or replace damaged heart tissue. Techniques such as patch grafts, where engineered cardiac tissue was applied to the infarcted area, have shown promise in improving heart function [ 15 ]. Biomaterials including natural polymers like fibrin and synthetic polymers like poly (glycerol sebacate) were explored in cardiac tissue engineering. Additionally, electrical stimulation was often used to enhance the maturation and functionality of the engineered cardiac tissue [ 16 ]. Liver tissue engineering seeks to develop functional liver tissues for transplantation and in vitro models for drug testing. Techniques involve the use of hepatocytes or stem cells seeded onto scaffolds. The goal was to create constructs that can perform essential liver functions. Advances include bioprinting liver tissues with precise architecture and incorporating vascular networks to support cell viability and function [ 17 ]. Nerve tissue engineering aims to repair peripheral nerve injuries and spinal cord damage. Techniques involve the use of scaffolds designed to guide axonal growth and support neural cell adhesion. Biomaterials such as poly (lactic-co-glycolic acid) (PLGA) and chitosan are commonly used, often combined with neurotrophic factors like nerve growth factor to promote nerve regeneration. Stem cells, including neural stem cells and MSCs, are also utilized for their potential to differentiate into neurons and glial cells. Clinical applications include the development of nerve conduits for bridging nerve gaps and enhancing functional recovery [ 18 ]. Tissue engineering also encompasses diverse applications such as corneal regeneration [ 19 ], ear cartilage engineering [ 20 ], and laryngeal tissue development [ 21 ], while dental pulp regeneration provides treatments for tooth decay and injury [ 22 ]. These examples demonstrate the broad scope and transformative potential of tissue engineering in medical interventions.
Biomaterials in tissue engineering
Introduction to biomaterials.
Biomaterials play a crucial role in tissue engineering, a field that combines engineering and biology to develop methods for replacing or regenerating human tissues. They are designed to provide an architectural framework reminiscent of native extracellular matrix (ECM) to encourage cell growth and eventual tissue regeneration [ 23 ]. An important avenue of tissue engineering is the development of biomaterials that can promote regenerative processes by effectively transporting cell populations and therapeutic agents, as well as providing structural scaffolding that confers sufficient mechanical properties to tissues. Moreover, the degradation rate of the biomaterial should ideally match the rate of new tissue formation at the implantation site, ensuring that the scaffold supports the regenerating tissue without leaving gaps or degrading prematurely [ 24 ]. Biomaterials have a long history of use in medical applications, such as intraocular lenses and dental fillings, but advances in cell and molecular biology, chemistry, materials science, and engineering have expanded their potential uses in clinical settings. Over the past 50 years, the definition of the ideal biomaterial has evolved significantly (Fig. 2 ). Initially, biomaterials were designed to be inert, matching the mechanical properties of native tissues and avoiding harmful host responses [ 25 ]. Early materials included bone cement, stainless steel, and Dacron, which were chosen for their mechanical properties and predictable foreign body responses. However, second-generation biomaterials, such as titanium, bioglass, PLGA, and collagen, were developed with bioactive properties like osseointegration, tissue integration, and biodegradation [ 26 ]. Hydrogels have been preferred for biomedical applications due to their excellent biocompatibility, adaptable structures, versatile synthesis methods, and favorable physical properties [ 27 , 28 ].
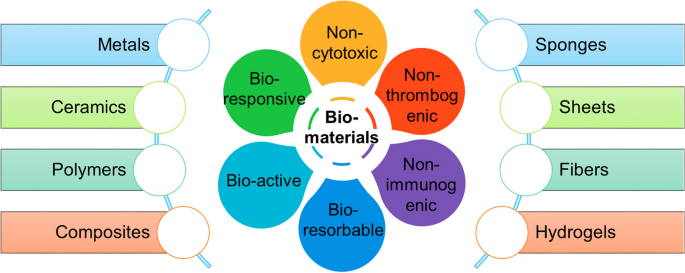
Schematic indicating the widely explored classes of biomaterials, popular forms of scaffolds and key characteristics of biomaterials
A wide range of biopolymers are being used to replace synthetic polymers across various industries, including biomedical [ 29 ]. Additionally, there are bio-based polymeric materials, like proteins, polysaccharides, and aliphatic polyesters, that are derived from plants, animals, or microbial synthesis, and offer eco-friendly approaches to enhancing sustainability [ 30 ]. These polymers differ from other biopolymers and can be either biodegradable or non-biodegradable [ 31 ]. Materials derived from biomass have minimal carbon footprints and possess multifunctional properties essential for promoting sustainability [ 32 ]. One such biomaterial was bacterial cellulose, which has been suggested for use in various biological applications, including wound healing, the production of artificial blood vessels, soft tissue engineering, and bone tissue engineering [ 33 ].
Trends in biomaterials
Currently, the focus is on biomaterials that integrate with adjacent tissues and enhance regenerative or reconstructive capacities. These materials are bioinductive, promoting the body's natural healing processes rather than merely being inert placeholders. The ECM is nature's template for biomaterials, providing support and scaffolding for cell growth. Scaffold materials in tissue engineering can be synthetic or naturally occurring [ 25 ]. Regardless of origin, they must support cell attachment, maintenance, proliferation, and sometimes differentiation while providing adequate structural support for the intended site. Additionally, the host response to these materials was critical for long-term success, especially in pediatric patients, where the scaffold must adapt to the growth and development of surrounding tissues [ 25 ]. Recent advancements in biomaterials for tissue engineering include the development of smart biomaterials that respond to external stimuli such as temperature, pH, magnetic, or electric fields. These materials offer dynamic properties that can better mimic natural tissue behavior. For instance, 3D and 4D bioprinting technologies have enabled the creation of complex, tissue-like structures that can change over time in response to environmental changes [ 34 ]. The clinical translation of tissue engineering strategies relies heavily on advancements in biomaterial development. While the application of these materials in pediatric patients remains limited, ongoing research aims to improve our understanding of the molecular environment during tissue regeneration, ultimately enhancing the effectiveness of tissue engineering approaches [ 25 ].
Critical role of biomaterials in tissue engineering
Biomaterials, that make scaffolds, play a critical role in tissue engineering, providing a platform for cell attachment and growth; therefore, the influence of scaffold characteristics on cell behavior is a key area of study [ 35 ]. Scaffolds direct the growth of cells seeded within the porous structure of the scaffold. Biomaterials significantly influence the fate of tissue engineering through their surface chemistry, mechanical properties, degradation profiles, and ability to deliver bioactive molecules [ 36 ]. Surface chemistry is critical as it affects protein adsorption, cell adhesion, and subsequent cell behavior. For instance, materials with specific cell-binding domains such as RGD (arginine-glycine-aspartic acid) can enhance cell adhesion, which is essential for tissue regeneration. Additionally, the surface modification of biomaterials with bioactive molecules like peptides and growth factors can further promote cell proliferation, migration, and differentiation [ 37 ]. The degradation rate of biomaterials is another vital aspect that influences tissue regeneration. An optimal degradation rate ensures that the scaffold provides adequate support for new tissue formation but degrades at a pace that does not impede the reconstruction process. Incorporating enzyme-sensitive peptides into biomaterials can control degradation rates, facilitating a balance between scaffold support and degradation [ 38 ].
Mechanical properties such as stiffness and elasticity also play a crucial role. They must match the native tissue's properties to support cell growth and tissue formation. For example, materials engineered for bone tissue engineering often include components like hydroxyapatite to provide the necessary mechanical strength [ 34 ]. The mechanical environment can influence cellular processes, including stem cell differentiation and tissue morphogenesis. Surface topology, including micro- and nanoscale patterning, can direct cellular responses even in the absence of biochemical signals [ 36 ]. Studies have shown that features such as particle shape and surface roughness can affect cell adhesion, proliferation, and differentiation. For instance, specific topographical cues can induce stem cells to differentiate into particular lineages, aiding in the development of functional tissues. Furthermore, the delivery of bioactive molecules through biomaterials is essential for guiding tissue regeneration [ 39 ]. Growth factors and peptides can be coupled with scaffolds to promote specific cellular activities. For example, biomaterials can be designed to release growth factors in a controlled manner to enhance angiogenesis and osteogenesis, vital for the regeneration of complex tissues such as bone and cartilage. The integration of biomaterials with the ECM components is also crucial. The ECM provides structural and biochemical support to surrounding cells, influencing cell behavior and tissue development. Biomaterials mimicking the ECM's properties can facilitate better integration with host tissues, promoting more effective tissue regeneration [ 40 ]. When a scaffold is implanted into the affected area, it should provide structural support for cell adhesion, proliferation, migration, and differentiation, promoting cellular activity similar to the in vivo environment. Additionally, it should allow for the controlled release of growth factors to regulate cell proliferation, viability, and differentiation [ 41 ].
Technologies in tissue engineering
Introduction to tissue engineering approaches.
Tissue engineering involves the fabrication of tissue constructs either in a bottom-up or top-down approach (Fig. 3 ). In the bottom-up approach , small building blocks such as cells, micro-tissues, or scaffolding materials are assembled to create more complex structures. This method focuses on the self-organization and self-assembly properties of cells and biomaterials, allowing for the precise design and fabrication of tissue constructs from the molecular or cellular level. Techniques like 3D bioprinting, layer-by-layer assembly, and the use of cell-laden hydrogels are common in bottom-up tissue engineering [ 42 ]. These techniques enable the creation of highly detailed and customizable tissue structures by placing cells and materials exactly where needed to mimic the natural architecture of tissues. 3D bioprinting, for example, involves the deposition of cell-laden bio-inks layer by layer to build complex tissue constructs. This technique allows for high precision and control over the placement of different cell types and materials, facilitating the creation of heterogeneous and functional tissues. The bottom-up approach also includes methods like organoid culture, where stem cells are guided to form miniaturized and simplified versions of organs, which can be used for disease modeling, drug testing, and regenerative medicine [ 43 ].
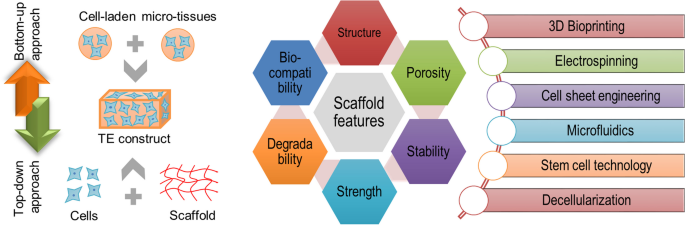
Schematic showing the bottom-up and top-down approaches, scaffold features that influence the overall cell response, and various popular technologies explored in tissue engineering
In contrast, the top-down approach starts with a pre-existing bulk material or scaffold, which is then shaped and modified to achieve the desired tissue structure. Techniques like electrospinning, freeze-drying, and molding are commonly used to create scaffolds with specific shapes and properties that support cell attachment, proliferation, and differentiation. Electrospinning, for instance, produces nanofibrous scaffolds that mimic the fibrous structure of natural ECM, providing a conducive environment for cell growth and tissue regeneration [ 44 ]. Freeze-drying can create porous scaffolds with high surface area, enhancing nutrient diffusion and cell infiltration. These top-down techniques leverage the inherent advantages of pre-existing materials and structures to guide tissue formation, often resulting in constructs that closely resemble the native tissue in terms of architecture and mechanical properties [ 45 ]. This approach also involves the use of decellularized tissues or organs, which retain the natural ECM architecture and mechanical properties of the original tissue [ 46 ]. The decellularized scaffold is then repopulated with patient-specific cells to regenerate functional tissues.
Both the bottom-up and the top-down methods have their unique advantages and challenges. The bottom-up approach allows for greater control over the microarchitecture and composition of the tissue, making it suitable for creating complex and heterogeneous structures. However, it can be limited by the difficulty in scaling up to larger tissue constructs. The top-down approach benefits from the use of natural scaffolds with inherent biological cues, but it may face challenges in achieving precise control over the microenvironment and integrating different cell types and materials.
Critical role of scaffold in tissue engineering
Scaffolds in tissue engineering are essential for directing cell behavior, tissue formation, and integration with host tissues. Their properties, such as porosity, mechanical strength, degradation rate, and surface characteristics, significantly influence the overall fate of the engineered tissue. Porosity is a key scaffold property that affects cell infiltration, nutrient diffusion, and waste removal [ 47 ]. Highly porous scaffolds provide ample space for cells to migrate and proliferate, which is essential for tissue regeneration. The size and interconnectedness of pores are crucial for vascularization, which supplies the engineered tissue with oxygen and nutrients. Optimal pore sizes vary depending on the type of tissue being engineered. For example, larger pores are typically beneficial for bone tissue engineering, while smaller pores are better suited for skin or cartilage regeneration. Mechanical strength and stiffness of the scaffold are also vital; scaffolds must mimic the mechanical properties of the target tissue to provide appropriate cues for cell differentiation For instance, softer scaffolds are preferred for neural or adipose tissues, whereas stiffer scaffolds are necessary for bone or cartilage tissues [ 48 ]. The mechanical properties influence mechano-transduction, where cells convert mechanical stimuli into biochemical signals, affecting gene expression and cell behavior. This property ensures that cells experience a similar environment to their native tissue, promoting proper tissue formation and function [ 49 ].
The degradation rate of the scaffold must be carefully controlled to match the rate of new tissue formation. A scaffold that degrades too quickly may not provide sufficient support for cells, while one that degrades too slowly can impede tissue integration and function. Biodegradable materials like polylactic acid (PLA) and polyglycolic acid are commonly used for their ability to break down into non-toxic byproducts, which are then absorbed or excreted by the body. The degradation products should not induce inflammation or toxicity, ensuring a safe environment for tissue regeneration [ 50 ]. Surface characteristics, including topography and chemical functionality, play a significant role in cell adhesion, proliferation, and differentiation. Surface modifications, such as coating with ECM proteins (e.g., collagen, fibronectin) or incorporating bioactive molecules (e.g., growth factors), can enhance cell-scaffold interactions [ 51 ]. Nanoscale features on the scaffold surface can mimic the natural ECM, providing cues that promote cell attachment and growth. Techniques like electrospinning can create nanofibrous scaffolds that replicate the fibrous nature of native tissues, supporting cellular behavior and tissue development [ 52 ].
Nanoscale scaffolds offer a higher surface area-to-volume ratio compared to microscale scaffolds, which can enhance cell adhesion and proliferation. Nanoscale features provide more precise topographical cues that mimic the natural ECM, promoting more natural cellular behaviours. Microscale scaffolds, however, can better facilitate cell infiltration and nutrient diffusion, which is crucial for the survival and function of larger tissue constructs. These scaffolds often support the formation of more substantial tissue masses and can be designed to include macroscopic features that aid in vascularization, a critical aspect of the viability of engineered tissues [ 53 ]. The interplay between these scaffold properties ultimately determines the success of the tissue engineering endeavour. By carefully designing and optimizing scaffold characteristics, researchers can create environments that closely mimic natural tissues, guiding cells toward the desired outcomes. This approach not only enhances the functional integration of engineered tissues but also improves the clinical applicability and success rates of tissue engineering therapies [ 54 ].
Technological trends in tissue engineering
Tissue engineering employs a variety of technologies to create functional tissues and organs, each with distinct methodologies and applications. One key technology is 3D bioprinting , which utilizes layer-by-layer deposition of bio-inks containing cells and biomaterials to fabricate complex tissue constructs. This technique allows for precise placement of cells and ECM components, enabling the creation of tissues with intricate architectures. For example, researchers have used 3D bioprinting to create skin grafts, cartilage, and vascularized tissues, demonstrating its potential for personalized medicine and complex tissue reconstruction [ 55 ]. Electrospinning is a technique used to produce nanofibrous scaffolds that mimic the fibrous structure of a natural ECM. These scaffolds provide a conducive environment for cell attachment, proliferation, and differentiation, making them suitable for applications in wound healing, nerve regeneration, and vascular grafts. Electrospun scaffolds have been successfully used to create artificial blood vessels and support the regeneration of peripheral nerves [ 56 ].
Microfluidics technology involves the manipulation of fluids at the microscale to create controlled microenvironments for cell culture and tissue engineering. Microfluidic devices can mimic the physiological conditions of tissues, allowing for the study of cell behaviors, drug testing, and the creation of tissue models. For instance, microfluidic chips have been used to create liver-on-a-chip models for drug toxicity testing and to develop vascularized tissue constructs for studying angiogenesis [ 57 ]. Organoid culture is a technology that uses stem cells to create miniaturized and simplified versions of organs in vitro. These organoids can recapitulate key aspects of organ development, function, and disease, providing valuable models for basic research, drug discovery, and regenerative medicine. Organoids have been developed for various tissues, including the brain, intestine, kidney, and liver, offering insights into tissue-specific physiology and pathology [ 58 ].
Stem cell technology is fundamental to tissue engineering, as it provides a source of cells capable of differentiating into various tissue types. Induced pluripotent stem cells and embryonic stem cells can be directed to form specific cell types and tissues, enabling the creation of patient-specific tissue constructs. Stem cell-derived tissues have been explored for applications in cardiac repair, spinal cord injury, and diabetes treatment [ 59 ]. Another important technology is decellularization , which involves removing cellular components from donor tissues or organs, leaving behind a scaffold composed of ECM. This scaffold retains native tissue architecture and mechanical properties, making it an ideal template for recellularization with patient-specific cells. Decellularized scaffolds have been used to engineer heart valves, lungs, and liver tissues, offering promising solutions for organ transplantation without the risk of immune rejection [ 60 ].
These technologies highlight innovative solutions for tissue repair and regeneration. The continuous advancement in these technologies holds promise for addressing the growing need for functional tissues and organs in regenerative medicine and transplantation.
Sustainability and sustainable development goals
Introduction to sustainability and sustainable development goals.
Sustainability is defined as development that meets the needs of the present without compromising the ability of future generations to meet their own needs. Adopted by all United Nations Member States in 2015 as part of the 2030 Agenda for Sustainable Development, the SDGs build on decades of work by countries and the UN, including the Millennium Development Goals. SDGs are a collection of 17 interlinked global goals designed to achieve a better and more sustainable future for all. The SDGs cover a broad range of social, economic, and environmental development issues and aim to address the global challenges we face, including poverty, inequality, climate change, environmental degradation, peace, and justice. They are unique in that they call for action by all countries, developed and developing, to promote prosperity while protecting the planet. Technically, the SDGs are grounded in a comprehensive set of 169 specific targets that are measurable and time-bound. Each goal has specific targets to be achieved over the next 15 years. The targets are designed to be monitored through a set of indicators developed by the Inter-Agency and Expert Group on SDG Indicators. A key feature of the SDGs is their universality. Technological innovation and digital transformation also play critical roles in advancing the SDGs. Partnerships and collaboration among governments, the private sector, civil society, and international organizations are crucial for the success of the SDGs. The goals explicitly acknowledge the need for multi-stakeholder partnerships to mobilize and share knowledge, expertise, technology, and financial resources.
Sustainability in healthcare
Healthcare is primarily addressed in SDG-3 "Ensure healthy lives and promote well-being for all at all ages". This goal encompasses a wide range of targets aimed at improving health outcomes, reducing mortality rates, and addressing global health challenges. SDG-3 includes 9 targets (Fig. 4 ) which aim to: (3.1) reduce the global maternal mortality ratio to less than 70 per 100,000 live births; (3.2) end preventable deaths of newborns and children under 5 years of age; (3.3) end the epidemics of AIDS, tuberculosis, malaria, and neglected tropical diseases, and combat hepatitis, water-borne diseases, and other communicable diseases; (3.4) reduce by one-third premature mortality from non-communicable diseases (NCDs); (3.5) strengthen the prevention and treatment of substance abuse; (3.6) halve the number of global deaths and injuries from road traffic accidents; (3.7) ensure universal access to sexual and reproductive health-care services, and the integration of reproductive health into national strategies and programs; (3.8) achieve universal health coverage, access to quality essential health-care services, and access to safe, effective, quality, and affordable essential medicines and vaccines for all; (3.9) substantially reduce the number of deaths and illnesses from hazardous chemicals and air, water, and soil pollution and contamination; and other means of implementation for the targets viz. (3a) strengthen the implementation of the World Health Organization Framework Convention on Tobacco Control in all countries, as appropriate; (3b) support the research and development of vaccines and medicines for the communicable and NCDs that primarily affect developing countries, provide access to affordable essential medicines and vaccines, and ensure that intellectual property rights do not undermine this goal, in line with the Doha Declaration; (3c) substantially increase health financing and the recruitment, development, training, and retention of the health workforce in developing countries, especially in least developed countries and small island developing states; and (3d) strengthen the capacity of all countries, particularly developing countries, for early warning, risk reduction, and management of national and global health risks.
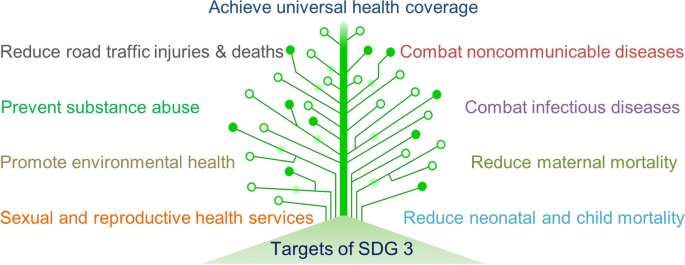
Schematic indicating the set of targets under ‘sustainable development goal 3 – good health and wellbeing’ as established by the United Nations
Sustainability in biomedical engineering
Biomedical engineering drives the creation of cutting-edge healthcare infrastructure, including advanced medical facilities equipped with state-of-the-art technology. These innovations not only improve patient outcomes but also stimulate economic growth through the development of a robust biomedical industry. Apart from contributing to SDG 3, the advancement of biomedical technologies also contributes to SDG 9, which focuses on building resilient infrastructure, promoting inclusive and sustainable industrialization, and fostering innovation. Biomedical engineering also intersects with SDG 12, which advocates for responsible consumption and production. And, the implementation of circular economy principles, where medical devices are designed for durability, reuse, and recyclability, also aligns with SDG 12. Sustainable design and manufacturing practices in the development of medical devices are crucial in minimizing environmental impact. Utilizing biodegradable materials for implants and reducing the use of hazardous substances in medical equipment production are steps toward achieving this goal. SDG 4, which focuses on quality education, is also relevant to biomedical engineering. Providing education and training in biomedical engineering not only equips individuals with the skills needed to innovate in the healthcare sector but also fosters a knowledgeable workforce capable of addressing complex health challenges. Finally, the pursuit of SDG 17, which emphasizes strengthening the means of implementation and revitalizing the global partnership for sustainable development, is essential in biomedical engineering. For example, public–private partnerships in biomedical research and development can lead to the creation of affordable healthcare solutions tailored to the needs of developing countries, addressing disparities in healthcare access and quality.
Sustainability in tissue engineering
Biomaterials such as polymers, metals, ceramics, composites, and decellularized materials, coupled with cutting-edge technologies like bioprinting, electrospinning, decellularization, cell sheet engineering, and microfluidics, are pivotal in advancing the SDGs. The innovations in biomaterials and tissue engineering technologies drive transformative changes across healthcare, environmental sustainability, and economic growth. By enabling precise tissue engineering, sustainable resource management, and enhanced medical treatments, biomaterials and associated technologies contribute significantly to global efforts aimed at achieving sustainable development and improving quality of life worldwide (Fig. 5 ).
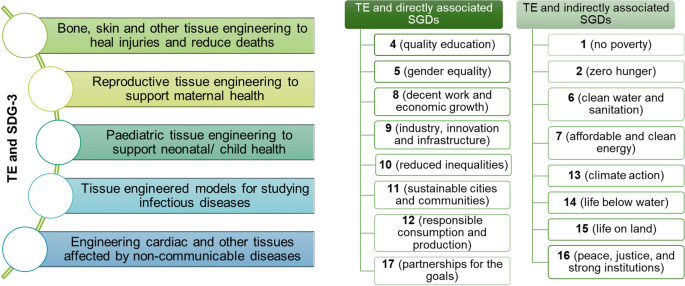
Schematic indicating how various aspects of tissue engineering align with SDG 3 in particular, and other SDGs via direct and indirect means
SDG-3 in tissue engineering
Biomaterials and tissue engineering have immense potential to transform healthcare and medical treatment, and therefore, contribute significantly towards SDG 3. For instance, a key target of SDG 3, is maternal mortality, which aims to reduce the global maternal mortality ratio to less than 70 per 100,000 live births by 2030. Various tissue engineering methods and biomaterials are being developed to support maternal health and reduce mortality, which also comes under target 1 of SDG 3. For instance, innovative biomimetic materials can be employed to create placenta-on-a-chip devices that simulate placental function, allowing for detailed pharmacokinetic studies and a better understanding of drug impacts during pregnancy [ 61 ]. Additionally, advancements in silk-based biomaterials have shown promise in treating cervical insufficiency, a condition that can lead to preterm birth and increased maternal mortality. These biomaterials, particularly hydrogel systems like alginate, collagen, and fibrin, provide a minimally invasive method to reinforce the cervix, reducing the risk of premature delivery. Biomaterials are being used to develop in vitro models that replicate the female reproductive system, providing crucial insights into conditions like preterm birth, which significantly contributes to maternal mortality [ 62 ]. These models allow researchers to study the physiological and pathological states of reproductive tissues under controlled conditions. Additionally, new hydrogel technologies are being investigated to address postpartum hemorrhage, a leading cause of maternal death. These hydrogels can be applied directly to bleeding sites to promote clotting and reduce blood loss effectively. Efforts to integrate biomaterials in clinical applications are further supported by a dedicated funding stream for women's health bioengineering, emphasizing the importance of targeted research and development in this field [ 63 ].
Advancements in biomaterials and tissue engineering hold significant promise for achieving SDG 3, specifically Target 2, which aims to end preventable deaths of newborns and children under five. In pediatric tissue engineering, biomaterial scaffolds have been developed to create functional environments conducive to tissue growth and repair. These scaffolds are crucial for neonatal and pediatric applications due to their ability to support cell growth, deliver drugs, and integrate with the host's biological systems, thereby reducing the risk of mortality in young patients. The field of tissue engineering has seen remarkable progress in managing congenital heart diseases, a leading cause of neonatal mortality [ 64 ]. Traditional treatments using synthetic grafts face challenges like thrombosis, infection, and limited growth potential. Tissue-engineered solutions, such as biodegradable scaffolds for vascular grafts, heart patches, and valves, offer a promising alternative. These scaffolds can grow and remodel within the patient, providing long-term solutions that adapt to the changing physiology of growing children [ 64 ].
The integration of biomaterials and tissue engineering methods offers promising strategies to combat infectious diseases, enhance therapeutic outcomes, and support the regeneration of damaged tissues, thus aligning with the goals of SDG 3, target 3. Engineered biomaterials play a crucial role in enhancing vaccine efficacy by stabilizing antigens, organizing antigen presentation, recruiting antigen-presenting cells, and delivering antigens to lymph nodes, thereby supporting and boosting effective immune responses. For instance, mesoporous silica rods have been developed for in situ modulation of host immune cells, showcasing their potential to create effective vaccines against infectious diseases [ 65 ]. Hydrogels, particularly injectable hydrogels, provide a versatile platform for delivering a wide variety of therapeutics due to their high biocompatibility, tunable physicochemical properties, and controlled release profiles. Moreover, biomaterials can be engineered to provide local immunomodulation, which is critical in managing infections by controlling immune responses and preventing tissue damage. Tissue engineering also contributes significantly to SDG 3 by promoting the regeneration of tissues damaged by infections [ 66 ].
Biomaterials and tissue engineering provide innovative solutions for treating and managing NCDs, such as cardiovascular diseases, diabetes, and musculoskeletal disorders, as part of SDG-3, target 4. For example, tissue-engineered vascular grafts and cardiac patches are being developed to repair damaged heart tissues and blood vessels, significantly improving the survival rates and quality of life for patients with cardiovascular diseases [ 67 ]. These advanced therapies offer regenerative solutions that traditional treatments cannot provide, addressing the root causes of tissue damage and promoting long-term health benefits. The development of biocompatible and biodegradable biomaterials enhances the effectiveness of medical implants and devices used in treating NCDs. For instance, bioresorbable stents made from materials like PLA are designed to dissolve after fulfilling their purpose, reducing complications associated with permanent implants [ 68 ]. Additionally, by using patient-specific cells and biomaterials, scientists can engineer tissues and organs tailored to the individual’s unique biological profile. This personalized approach enhances the effectiveness of treatments, reduces the likelihood of rejection, and accelerates recovery times [ 69 ].
Tissue engineering technology is being adopted to restore reproductive functions affected by disorders such as infertility and sexual dysfunction. Biomaterial-based drug delivery systems are being designed to provide controlled and sustained release of contraceptives, enhancing their effectiveness and convenience. Biomaterials like alginate, collagen, and hyaluronic acid are being extensively used in facilitating in vitro follicle culture systems that restore fertility for female patient’s post-cancer treatments [ 70 ]. Advanced biomedical engineering technologies, including microfluidic chips and 3D printing, have been developed to address reproductive health challenges. These technologies offer precise control over the reproductive microenvironment, improving outcomes in assisted reproductive technology and contraception [ 71 ]. Biomaterial-based scaffolds and implants are designed to provide structural support and promote the regeneration of pelvic floor tissues, offering minimally invasive treatment options that improve health outcomes and recovery times [ 72 ]. This integration aligns with the goals of SDG 3, Target 7, promoting well-being and healthy lives for all ages.
Biomaterials and tissue engineering technologies could also contribute to other targets of SDG3. For instance, tissue-engineered skin grafts and bone scaffolds can significantly improve recovery outcomes for accident victims by promoting faster and more effective healing of severe wounds and fractures [ 73 ]. These technologies enhance trauma care and reduce the long-term impact of injuries, and thus contribute towards target 6 of SDG-3. The development of cost-effective biomaterials and scalable tissue engineering technologies can make advanced medical treatments more accessible and affordable. These innovations contribute to achieving universal health coverage by providing high-quality, affordable medical solutions, part of target 8 of SDG-3. Tissue-engineered models of the liver and lungs can be used to study the toxicological impacts of pollutants and develop strategies to reduce exposure and harm [ 74 ]. These models enable more accurate assessments of how pollutants affect human tissues, leading to better regulatory policies and protective measures; and thus, contribute towards target 9 of SDG-3. An indicative list of how tissue engineering aligns with specific targets under SDG 3 (Good Health and Well-being) is presented in Table 1 .
Associated SDGs in tissue engineering
Biomaterials and technologies in tissue engineering are intricately linked with several other SDGs. For instance, tissue engineering contributes to SDG 4 (Quality Education) by enhancing educational experiences through innovative learning tools like bioprinted tissues and organ models, providing hands-on experience and a deeper understanding of human anatomy and disease mechanisms [ 77 ]. Promoting gender equality (SDG 5) in tissue engineering involves initiatives like targeted scholarships, mentorship programs, and inclusive policies to support women's participation and professional growth in STEM fields. Towards SDG 8 (Decent Work and Economic Growth), the tissue engineering sector drives economic growth and job creation across biotechnology, pharmaceuticals, and healthcare, with various companies generating high-skilled employment opportunities [ 6 ]. As part of SDG 9 (Industry, Innovation, and Infrastructure), tissue engineering fosters industry innovation through advanced technologies like 3D bioprinting, electrospinning, and microfluidics, and establishes robust research infrastructure via partnerships between academia, private companies, and government agencies [ 78 ].
Besides, developing cost-effective biomaterials and scalable technologies ensures that advanced medical treatments are accessible in low- and middle-income countries, reducing health disparities and promoting equitable healthcare; therefore, contributing towards SDG 10 (Reduced Inequality). As part of SDG 11 (Sustainable Cities and Communities), minimizing medical waste by using biodegradable biomaterials, using bioprinting technologies to mass produce tissues for highly demanding urban populations, integration of smart technologies contributes to the development of sustainable healthcare system in urban settings [ 79 ]. Further, the use of renewable and biodegradable materials in tissue engineering, such as silk fibroin from seri-farming, reduces environmental impact and aligns with SDG 12 (Responsible Consumption and Production) [ 80 ]. Last but not least, achieving SDGs through tissue engineering relies on strong partnerships (SDG 17) across academia, industry, government, and NGOs, with initiatives like the tissue engineering and Regenerative Medicine International Society fostering global collaboration and knowledge exchange [ 81 ].
Other SDGs in tissue engineering
By advancing medical science and promoting sustainable practices, biomaterials and technologies in tissue engineering contribute to the broader objectives of sustainable development in various indirect ways, including SDG 1 (No Poverty), SDG 2 (Zero Hunger), SDG 6 (Clean Water and Sanitation), SDG 7 (Affordable and Clean Energy), SDG 13 (Climate Action), SDG 14 (Life Below Water), SDG 15 (Life on Land), and SDG 16 (Peace, Justice, and Strong Institutions). For instance, sustainable practices in the production and disposal of biomaterials and tissue engineering products help minimize water usage, pollution including micro-plastics, and overall environmental impact. The development of sustainable biomaterials and tissue engineering technologies with low carbon footprints contributes to climate change mitigation. Biomaterials derived from marine organisms, such as chitosan from crustacean shells, are used in tissue engineering applications, supporting sustainable resource utilization and reducing waste [ 82 ]. These practices help protect marine ecosystems and promote the sustainable use of ocean resources. Tissue engineering technologies promote the sustainable use of terrestrial ecosystems; for instance, silk fibroin from silk cocoons can be used in applications such as tissue scaffolds for regenerative medicine, contributing to the conservation of biodiversity and the sustainable use of land-based resources [ 80 ]. Lastly, by ensuring that advanced medical treatments are accessible and affordable to all, including marginalized and low-income populations, tissue engineering promotes social equity and justice.
Challenges and future outlook
The future direction of tissue engineering is poised to be defined by its capability to integrate both technological advancements and sustainable practices, which is especially critical in the realm of UN SDGs. Tissue engineering has historically focused on three core elements viz. cell-based, growth factor-based, and scaffold-based approaches. However, with increasing awareness of environmental impacts and medical innovation, future trends must address both functional medical requirements and sustainability goals.
However, implementing sustainability principles in tissue engineering to comply with UN SDGs faces several challenges. For instance, sourcing eco-friendly and sustainable biomaterials that maintain quality and biocompatibility is challenging, as many traditional materials are derived from non-renewable resources or involve energy-intensive processes. Next, sustainable practices, including using green materials and processes, often have higher initial costs, making it difficult for tissue engineering solutions to be economically competitive. Next, techniques like 3D printing, scaffold fabrication, and sterilization processes are energy-intensive, posing challenges in reducing the carbon footprint without compromising the quality of engineered tissues. Next, developing effective waste management strategies for bio-based and synthetic materials, including disposal of laboratory waste and chemical residues, is a challenge. Next, scaling up sustainable tissue engineering processes while ensuring reproducibility and standardization can be complex, particularly when using diverse and innovative materials. Next, meeting both sustainability standards and regulatory requirements for clinical use is demanding, as guidelines for eco-friendly practices in the biomedical sector are still evolving. Next, conducting comprehensive life cycle assessment to evaluate the environmental impact of tissue-engineered products throughout their lifespan is challenging due to the complexity of manufacturing processes and the lack of standardized metrics. Next, sustainability in tissue engineering requires collaboration between biologists, engineers, environmental scientists, and economists, which can be challenging to coordinate effectively. Further, there is lack of awareness and training on sustainability practices among researchers, manufacturers, and healthcare professionals within the tissue engineering field. Lastly, convincing stakeholders, including clinicians, industry partners, and the public, to adopt sustainable practices is challenging.
Yet, these challenges in implementing sustainability in tissue engineering present several opportunities and a positive future outlook. First, advancing bio-based polymers, recycled materials, and upcycled biowaste offers eco-friendly alternatives without compromising biocompatibility. Next, automated fabrication, 3D bioprinting, and scalable bioreactor systems can reduce costs, making sustainable products more economically viable. Next, integrating renewable energy and low-energy techniques can significantly reduce the carbon footprint of tissue-engineered products. Next, biodegradable scaffolds, closed-loop systems, and chemical recycling can enhance waste management, leading to sustainable laboratory practices. Next, establishing clearer guidelines and international standards for sustainable tissue engineering will facilitate compliance and clinical translation. Next, robust life cycle assessment tools tailored for biomedical products will help optimize processes and minimize environmental impact. Next, increased collaboration among diverse experts can drive innovations balancing sustainability and biomedical efficacy. Next, expanding training and educational programs will create a new generation of researchers skilled in sustainable practices. Next, rising demand for eco-friendly medical products can drive industry investments in sustainability and create market advantages. Lastly, emphasizing reuse, recycling, and upcycling of materials aligns tissue engineering with circular economy principles, reducing waste and resource consumption.
In summary, tissue engineering has evolved to incorporate a wide range of biomaterials such as polymers, metals, ceramics, composites, and decellularized materials. These materials are engineered to mimic the ECM, providing structural and functional support for cell growth and tissue regeneration. Technologies such as bioprinting, electrospinning, decellularization, cell sheet engineering, and microfluidics have further enhanced the precision and efficiency of creating complex tissue constructs. The integration of these technologies with sustainable practices pave the way for environmentally friendly and ethically responsible medical solutions. The alignment of tissue engineering with SDG 3 is evident through the development of advanced medical treatments that improve health outcomes and accessibility. Additionally, the field's contributions to SDGs 4, 5, 8, 9, 10, 11, 12 and 17 highlight its broader impact on social and economic development. Furthermore, tissue engineering indirectly supports SDGs 1, 2, 6, 7, 13, 14, 15 and 16 by promoting sustainable practices, reducing environmental impact, and fostering inclusive opportunities. As tissue engineering continues to evolve, the emphasis on affordability and accessibility, especially in low-resource settings, will ensure that the field not only meets medical needs but also fosters social equity. Overall, tissue engineering represents a transformative domain that can achieve sustainable healthcare solutions, advancing both human health and environmental stewardship.
Data availability
No datasets were generated or analysed during the current study.
https://sdgs.un.org/goals , (n.d.). https://sdgs.un.org/goals .
Langer R, Vacanti JP. Tissue engineering. Science. 1993;260:920–6. https://doi.org/10.1126/science.8493529 .
Article CAS PubMed Google Scholar
Vacanti CA. The history of tissue engineering. J Cell Mol Med. 2006;10:569–76. https://doi.org/10.1111/j.1582-4934.2006.tb00421.x .
Article PubMed Google Scholar
Vacanti CA, Vacanti JP. Functional organ replacement, the new technology of tissue engineering. Surg Technol Int. 1991;1:43–9.
Google Scholar
O’Connell CD, Duchi S, Onofrillo C, Caballero-Aguilar LM, Trengove A, Doyle SE, Zywicki WJ, Pirogova E, Di Bella C. Within or without you? A perspective comparing in situ and ex situ tissue engineering strategies for articular cartilage repair. Adv Healthc Mater. 2022;11:2201305. https://doi.org/10.1002/adhm.202201305 .
Article CAS PubMed PubMed Central Google Scholar
Jaklenec A, Stamp A, Deweerd E, Sherwin A, Langer R. Progress in the tissue engineering and stem cell industry “are we there yet?” Tissue Eng Part B Rev. 2012;18:155–66. https://doi.org/10.1089/ten.teb.2011.0553 .
Engler AJ, Sen S, Sweeney HL, Discher DE. Matrix elasticity directs stem cell lineage specification. Cell. 2006;126:677–89. https://doi.org/10.1016/j.cell.2006.06.044 .
Rouwkema J, Gibbs S, Lutolf MP, Martin I, Vunjak-Novakovic G, Malda J. In vitro platforms for tissue engineering: implications for basic research and clinical translation. J Tissue Eng Regen Med. 2011;5:e164–7. https://doi.org/10.1002/term.414 .
Chang H-I, Wang Y. Cell Responses to Surface and Architecture of Tissue Engineering Scaffolds, in: D. Eberli (Ed.), Regen. Med. Tissue Eng. - Cells Biomater., InTech, 2011. https://doi.org/10.5772/21983 .
Howard D, Buttery LD, Shakesheff KM, Roberts SJ. Tissue engineering: strategies, stem cells and scaffolds. J Anat. 2008;213:66–72. https://doi.org/10.1111/j.1469-7580.2008.00878.x .
Jorgensen AM, Mahajan N, Atala A, Murphy SV. Advances in skin tissue engineering and regenerative medicine. J Burn Care Res. 2023;44:S33–41. https://doi.org/10.1093/jbcr/irac126 .
Jaiswal C, Chandra V, Pant KK. skin tissue engineering: a review. J Adv Sci Res. 2022;13:01–11. https://doi.org/10.55218/JASR.202213401 .
Article Google Scholar
Gayanthry G, Athira RK, Anju MS, PR AK, PR HV, Kasoju N, Komath M. Mesenchymal stem cell culture in aligned porous hydroxyapatite scaffolds using a multiwell plate bioreactor for bone tissue engineering. MedComm - Future Med. 2022;1:e17. https://doi.org/10.1002/mef2.17 .
Ansari M. Bone tissue regeneration: biology, strategies and interface studies. Prog Biomater. 2019;8:223–37. https://doi.org/10.1007/s40204-019-00125-z .
Ye KY, Black LD. Strategies for tissue engineering cardiac constructs to affect functional repair following myocardial infarction. J Cardiovasc Transl Res. 2011;4:575. https://doi.org/10.1007/s12265-011-9303-1 .
Article PubMed PubMed Central Google Scholar
Chiu LLY. Cardiac tissue engineering: current state and perspectives. Front Biosci. 2012;17:1533. https://doi.org/10.2741/4002 .
Article CAS Google Scholar
Ming Z, Tang X, Liu J, Ruan B. Advancements in research on constructing physiological and pathological liver models and their applications utilizing bioprinting technology. Molecules. 2023;28:3683. https://doi.org/10.3390/molecules28093683 .
Yi S, Xu L, Gu X. Scaffolds for peripheral nerve repair and reconstruction. Exp Neurol. 2019;319:112761. https://doi.org/10.1016/j.expneurol.2018.05.016 .
Beena M, Ameer JM, Kasoju N. Optically clear silk fibroin films with tunable properties for potential corneal tissue engineering applications: a process–property–function relationship study. ACS Omega. 2022;7:29634–46. https://doi.org/10.1021/acsomega.2c01579 .
Xue J, Feng B, Zheng R, Lu Y, Zhou G, Liu W, Cao Y, Zhang Y, Zhang WJ. Engineering ear-shaped cartilage using electrospun fibrous membranes of gelatin/polycaprolactone. Biomaterials. 2013;34:2624–31. https://doi.org/10.1016/j.biomaterials.2012.12.011 .
Hamilton NJI, Birchall MA. Tissue-engineered larynx: future applications in laryngeal cancer. Curr Otorhinolaryngol Rep. 2017;5:42–8. https://doi.org/10.1007/s40136-017-0144-6 .
Demarco FF, Conde MCM, Cavalcanti BN, Casagrande L, Sakai VT, Nör JE. Dental pulp tissue engineering. Braz Dent J. 2011;22:3–13. https://doi.org/10.1590/S0103-64402011000100001 .
Lee EJ, Kasper FK, Mikos AG. Biomaterials for tissue engineering. Ann Biomed Eng. 2014;42:323–37. https://doi.org/10.1007/s10439-013-0859-6 .
Abbott RD, Kaplan DL. Engineering biomaterials for enhanced tissue regeneration. Curr Stem Cell Rep. 2016;2:140–6. https://doi.org/10.1007/s40778-016-0039-3 .
Keane TJ, Badylak SF. Biomaterials for tissue engineering applications. Semin Pediatr Surg. 2014;23:112–8. https://doi.org/10.1053/j.sempedsurg.2014.06.010 .
Navarro M, Michiardi A, Castaño O, Planell JA. Biomaterials in orthopaedics. J R Soc Interface. 2008;5:1137–58. https://doi.org/10.1098/rsif.2008.0151 .
Hu H, Xu F-J. Rational design and latest advances of polysaccharide-based hydrogels for wound healing. Biomater Sci. 2020;8:2084–101. https://doi.org/10.1039/D0BM00055H .
Fu L-H, Qi C, Ma M-G, Wan P. Multifunctional cellulose-based hydrogels for biomedical applications. J Mater Chem B. 2019;7:1541–62. https://doi.org/10.1039/C8TB02331J .
Arif ZU, Khalid MY, Sheikh MF, Zolfagharian A, Bodaghi M. Biopolymeric sustainable materials and their emerging applications. J Environ Chem Eng. 2022;10:108159. https://doi.org/10.1016/j.jece.2022.108159 .
Beena Unni A, Muringayij Joseph T. Enhancing polymer sustainability: eco-conscious strategies. Polymers. 2024;16:1769. https://doi.org/10.3390/polym16131769 .
Arif ZU, Khalid MY, Noroozi R, Hossain M, Shi HH, Tariq A, Ramakrishna S, Umer R. Additive manufacturing of sustainable biomaterials for biomedical applications. Asian J Pharm Sci. 2023;18:100812. https://doi.org/10.1016/j.ajps.2023.100812 .
Khalid MY, Arif ZU, Al Rashid A, Bukhari SM, Hossain M, Koç M. Shape-memory and self-healing properties of sustainable cellulosic nanofibers-based hybrid materials for novel applications. Giant. 2024;19:100299. https://doi.org/10.1016/j.giant.2024.100299 .
Qian H, Liu J, Wang X, Pei W, Fu C, Ma M, Huang C. The state-of-the-art application of functional bacterial cellulose-based materials in biomedical fields. Carbohydr Polym. 2023;300:120252. https://doi.org/10.1016/j.carbpol.2022.120252 .
Eldeeb AE, Salah S, Elkasabgy NA. Biomaterials for tissue engineering applications and current updates in the field: a comprehensive review. AAPS PharmSciTech. 2022;23:267. https://doi.org/10.1208/s12249-022-02419-1 .
Khang G, Lee SJ, Kim MS, Lee HB. Biomaterials: Tissue Engineering and Scaffolds, in: J.G. Webster (Ed.), Encycl. Med. Devices Instrum., 1st ed., Wiley, 2006. https://doi.org/10.1002/0471732877.emd029 .
Kim HS, Kumbar SG, Nukavarapu SP. Biomaterial-directed cell behavior for tissue engineering. Curr Opin Biomed Eng. 2021;17:100260. https://doi.org/10.1016/j.cobme.2020.100260 .
Mobasseri R, Tian L, Soleimani M, Ramakrishna S, Naderi-Manesh H. Bio-active molecules modified surfaces enhanced mesenchymal stem cell adhesion and proliferation. Biochem Biophys Res Commun. 2017;483:312–7. https://doi.org/10.1016/j.bbrc.2016.12.146 .
Umuhoza D, Yang F, Long D, Hao Z, Dai J, Zhao A. Strategies for tuning the biodegradation of silk fibroin-based materials for tissue engineering applications. ACS Biomater Sci Eng. 2020;6:1290–310. https://doi.org/10.1021/acsbiomaterials.9b01781 .
Nava MM, Raimondi MT, Pietrabissa R. Controlling self-renewal and differentiation of stem cells via mechanical cues. J Biomed Biotechnol. 2012;2012:1–12. https://doi.org/10.1155/2012/797410 .
Chen C, Bang S, Cho Y, Lee S, Lee I, Zhang S, Noh I. Research trends in biomimetic medical materials for tissue engineering: 3D bioprinting, surface modification, nano/micro-technology and clinical aspects in tissue engineering of cartilage and bone. Biomater Res. 2016;20:10. https://doi.org/10.1186/s40824-016-0057-3 .
Arif ZU, The role of polysaccharide-based biodegradable soft polymers in the healthcare sector, Adv. Ind. Eng. Polym. Res. 2024;S2542504824000241. https://doi.org/10.1016/j.aiepr.2024.05.001 .
Anupama Sekar J, Athira RK, Lakshmi TS, Velayudhan S, Bhatt A, Anil Kumar PR, Kasoju N. 3D Bioprinting in Tissue Engineering and Regenerative Medicine: Current Landscape and Future Prospects, in: B. Bhaskar, P. Sreenivasa Rao, N. Kasoju, V. Nagarjuna, R.R. Baadhe (Eds.), Biomater. Tissue Eng. Regen. Med., Springer Singapore, Singapore, 2021: pp. 561–580. https://doi.org/10.1007/978-981-16-0002-9_17 .
Gong P, Zheng W, Xiao D, Jiang X. Microscale methods to assemble mammalian cells into tissue-like structures. Sci China Life Sci. 2012;55:862–71. https://doi.org/10.1007/s11427-012-4385-9 .
Kasoju N, Ye H, Cui Z, Ramakrishna S. Electrospinning and electrospraying in biomedical engineering, in: Biomed Appl Electrospinning Electrospraying, Elsevier, 2021: pp. 375–393. https://doi.org/10.1016/B978-0-12-822476-2.00015-7 .
Hou Q, Grijpma DW, Feijen J. Preparation of interconnected highly porous polymeric structures by a replication and freeze-drying process. J Biomed Mater Res B Appl Biomater. 2003;67B:732–40. https://doi.org/10.1002/jbm.b.10066 .
Rowley AT, Nagalla RR, Wang S, Liu WF. Extracellular matrix-based strategies for immunomodulatory biomaterials engineering. Adv Healthc Mater. 2019;8:1801578. https://doi.org/10.1002/adhm.201801578 .
Bružauskaitė I, Bironaitė D, Bagdonas E, Bernotienė E. Scaffolds and cells for tissue regeneration: different scaffold pore sizes—different cell effects. Cytotechnology. 2016;68:355–69. https://doi.org/10.1007/s10616-015-9895-4 .
Leipzig ND, Shoichet MS. The effect of substrate stiffness on adult neural stem cell behavior. Biomaterials. 2009;30:6867–78. https://doi.org/10.1016/j.biomaterials.2009.09.002 .
J.H. Lee, J. Kisiday, A.J. Grodzinsky, Tissue‐Engineered Versus Native Cartilage: Linkage between Cellular Mechano‐Transduction and Biomechanical Properties, in: G. Bock, J. Goode (Eds.), Novartis Found. Symp., 1st ed., Wiley, 2003: pp. 52–69. https://doi.org/10.1002/0470867973.ch5 .
Singhal AR, Agrawal CM, Athanasiou KA. Salient degradation features of a 50:50 PLA/PGA scaffold for tissue engineering. Tissue Eng. 1996;2:197–207. https://doi.org/10.1089/ten.1996.2.197 .
Bacakova L, Filova E, Parizek M, Ruml T, Svorcik V. Modulation of cell adhesion, proliferation and differentiation on materials designed for body implants. Biotechnol Adv. 2011;29:739–67. https://doi.org/10.1016/j.biotechadv.2011.06.004 .
Murugan R, Ramakrishna S. Nano-featured scaffolds for tissue engineering: a review of spinning methodologies. Tissue Eng. 2006;12:435–47. https://doi.org/10.1089/ten.2006.12.435 .
Ng R, Zang R, Yang KK, Liu N, Yang S-T. Three-dimensional fibrous scaffolds with microstructures and nanotextures for tissue engineering. RSC Adv. 2012;2:10110. https://doi.org/10.1039/c2ra21085a .
Leong K, Chua C, Sudarmadji N, Yeong W. Engineering functionally graded tissue engineering scaffolds. J Mech Behav Biomed Mater. 2008;1:140–52. https://doi.org/10.1016/j.jmbbm.2007.11.002 .
Tan B, Gan S, Wang X, Liu W, Li X. Applications of 3D bioprinting in tissue engineering: advantages, deficiencies, improvements, and future perspectives. J Mater Chem B. 2021;9:5385–413. https://doi.org/10.1039/D1TB00172H .
Xie X, Chen Y, Wang X, Xu X, Shen Y, Khan AUR, Aldalbahi A, Fetz AE, Bowlin GL, El-Newehy M, Mo X. Electrospinning nanofiber scaffolds for soft and hard tissue regeneration. J Mater Sci Technol. 2020;59:243–61. https://doi.org/10.1016/j.jmst.2020.04.037 .
Bahrami S, Baheiraei N, Najafi-Ashtiani M, Nour S, Razavi M, Microfluidic devices in tissue engineering, in: Biomed. Appl. Microfluid. Devices, Elsevier, 2021: pp. 209–233. https://doi.org/10.1016/B978-0-12-818791-3.00008-5 .
Zhang Y, Organoids As a Tool to Fuel Basic Medical Research. J Complement Med Altern Healthc 2023;11. https://doi.org/10.19080/JCMAH.2023.11.555823 .
Hunt NC, Lako M. Tissue engineering using pluripotent stem cells: multidisciplinary approaches to accelerate bench-to-bedside transition. Regen Med. 2016;11:495–8. https://doi.org/10.2217/rme-2016-0095 .
Fu R-H, Wang Y-C, Liu S-P, Shih T-R, Lin H-L, Chen Y-M, Sung J-H, Lu C-H, Wei J-R, Wang Z-W, Huang S-J, Tsai C-H, Shyu W-C, Lin S-Z. Decellularization and recellularization technologies in tissue engineering. Cell Transpl. 2014;23:621–30. https://doi.org/10.3727/096368914X678382 .
Elzinga FA, Khalili B, Touw DJ, Prins JR, Olinga P, Leuvenink HGD, van Goor H, Gordijn SJ, Nagelkerke A, Mian P. Placenta-on-a-chip as an in vitro approach to evaluate the physiological and structural characteristics of the human placental barrier upon drug exposure: a systematic review. J Clin Med. 2023;12:4315. https://doi.org/10.3390/jcm12134315 .
Gargus ES, Rogers HB, McKinnon KE, Edmonds ME, Woodruff TK. Engineered reproductive tissues. Nat Biomed Eng. 2020;4:381–93. https://doi.org/10.1038/s41551-020-0525-x .
Fogg K, Tseng N-H, Peyton SR, Holeman P, Mc Loughlin S, Fisher JP, Sutton A, Shikanov A, Gnecco JS, Knight KM, Slaby EM, Weaver JD, Hashemi NN, Zhang Y, House MD, Vogt BJ, Aguado BA, Bradford JC, Robinson JL, Thomas PK, Lau AG, Oyen ML. Roadmap on biomaterials for women’s health. J Phys Mater. 2023;6:012501. https://doi.org/10.1088/2515-7639/ac90ee .
Blum KM, Mirhaidari GJM, Breuer CK. Tissue engineering: Relevance to neonatal congenital heart disease. Semin Fetal Neonatal Med. 2022;27:101225. https://doi.org/10.1016/j.siny.2021.101225 .
Dellacherie MO, Li A, Lu BY, Verbeke CS, Gu L, Stafford AG, Doherty EJ, Mooney DJ. Single-shot mesoporous silica rods scaffold for induction of humoral responses against small antigens. Adv Funct Mater. 2020;30:2002448. https://doi.org/10.1002/adfm.202002448 .
Zarubova J, Zhang X, Hoffman T, Hasani-Sadrabadi MM, Li S. Biomaterial-based immunoengineering to fight COVID-19 and infectious diseases. Matter. 2021;4:1528–54. https://doi.org/10.1016/j.matt.2021.02.025 .
Dogan A, Eser Elcin A, Murat EY. Translational applications of tissue engineering in cardiovascular medicine. Curr Pharm Des. 2017;23:903–14. https://doi.org/10.2174/1381612823666161111141954 .
Waksman R. The disappearing stent: when plastic replaces metal. Circulation. 2012;125:2291–4. https://doi.org/10.1161/CIRCULATIONAHA.112.103911 .
Neves LS, Rodrigues MT, Reis RL, Gomes ME. Current approaches and future perspectives on strategies for the development of personalized tissue engineering therapies. Expert Rev Precis Med Drug Dev. 2016;1:93–108. https://doi.org/10.1080/23808993.2016.1140004 .
Desai N, Alex A, AbdelHafez F, Calabro A, Goldfarb J, Fleischman A, Falcone T. Three-dimensional in vitro follicle growth: overview of culture models, biomaterials, design parameters and future directions. Reprod Biol Endocrinol. 2010;8:119. https://doi.org/10.1186/1477-7827-8-119 .
Wang X, Wu D, Li W, Yang L. Emerging biomaterials for reproductive medicine. Eng Regen. 2021;2:230–45. https://doi.org/10.1016/j.engreg.2021.11.006 .
Mukherjee S, Darzi S, Paul K, Werkmeister JA, Gargett CE. Mesenchymal stem cell-based bioengineered constructs: foreign body response, cross-talk with macrophages and impact of biomaterial design strategies for pelvic floor disorders. Interface Focus. 2019;9:20180089. https://doi.org/10.1098/rsfs.2018.0089 .
Colazo JM, Evans BC, Farinas AF, Al-Kassis S, Duvall CL, Thayer WP. Applied bioengineering in tissue reconstruction, replacement, and regeneration. Tissue Eng Part B Rev. 2019;25:259–90. https://doi.org/10.1089/ten.teb.2018.0325 .
Nichols JE, Niles JA, Vega SP, Argueta LB, Eastaway A, Cortiella J. Modeling the lung: Design and development of tissue engineered macro- and micro-physiologic lung models for research use. Exp Biol Med. 2014;239:1135–69. https://doi.org/10.1177/1535370214536679 .
Maughan E, Lesage F, Butler CR, Hynds RE, Hewitt R, Janes SM, Deprest JA, Coppi PD. Airway tissue engineering for congenital laryngotracheal disease. Semin Pediatr Surg. 2016;25:186–90. https://doi.org/10.1053/j.sempedsurg.2016.02.012 .
Ali ASM, Berg J, Roehrs V, Wu D, Hackethal J, Braeuning A, Woelken L, Rauh C, Kurreck J. Xeno-Free 3D bioprinted liver model for hepatotoxicity assessment. Int J Mol Sci. 2024;25:1811. https://doi.org/10.3390/ijms25031811 .
Singh G, Mohapatra M. Three-dimensional printing, bioink, organ printing, and tissue engineering technologies, and their application in modern anatomical pedagogy. Natl J Clin Anat. 2023;12:223–6. https://doi.org/10.4103/NJCA.NJCA_182_23 .
Ashammakhi N, GhavamiNejad A, Tutar R, Fricker A, Roy I, Chatzistavrou X, Hoque Apu E, Nguyen K-L, Ahsan T, Pountos I, Caterson EJ. Highlights on advancing frontiers in tissue engineering. Tissue Eng Part B Rev. 2022;28:633–64. https://doi.org/10.1089/ten.teb.2021.0012 .
Díaz Lantada A, De Maria C. Sustainable open-source medical devices manufactured with green biomaterials and accessible resources. Curr Opin Biomed Eng. 2023;28:100500. https://doi.org/10.1016/j.cobme.2023.100500 .
Kasoju N, Bora U. Silk fibroin in tissue engineering. Adv Healthc Mater. 2012;1:393–412. https://doi.org/10.1002/adhm.201200097 .
Bertram TA, Johnson PC, Tawil BJ, Van Dyke M, Hellman KB. Enhancing tissue engineering and regenerative medicine product commercialization: the role of science in regulatory decision-making for the TE/RM product development. Tissue Eng Part A. 2015;21:2476–9. https://doi.org/10.1089/ten.tea.2015.0136 .
Omoregie IP, Osagie AD, Ogochukwu OO. Crustacean nanochitosan-based bioremediation of nanoplastic-polluted aquatic habitat: a review pursuant to SDG 6. Sci Afr. 2023;21:e01881. https://doi.org/10.1016/j.sciaf.2023.e01881 .
Download references
Acknowledgements
Authors acknowledge the support from SCTIMST Trivandrum and Department of Science and Technology (GOI).
Department of Science and Technology, Ministry of Science and Technology, Government of India through Technical Research Centre for Biomedical Devices (TRC-P8311) .
Author information
Authors and affiliations.
Division of Tissue Culture, Department of Applied Biology, Biomedical Technology Wing, Sree Chitra Tirunal Institute for Medical Sciences and Technology, Thiruvananthapuram, 695012, Kerala, India
Naresh Kasoju & Anagha Sunilkumar
You can also search for this author in PubMed Google Scholar
Contributions
NK - conceptualization, formal analysis, writing - review and editing, visualization, funding acquisition, project administration, resources, supervision. AS – methodology, data curation, writing - original draft. All authors read and approved the final manuscript.
Corresponding author
Correspondence to Naresh Kasoju .
Ethics declarations
Ethics approval and consent to participate.
Not applicable, as it does not involve the use of any animal or human data or tissue.
Consent for publication
Not applicable, as it does not contain any individual person’s data in any form.
Competing interests
The authors declare no competing interests.
Additional information
Publisher’s note.
Springer Nature remains neutral with regard to jurisdictional claims in published maps and institutional affiliations.
Rights and permissions
Open Access This article is licensed under a Creative Commons Attribution-NonCommercial-NoDerivatives 4.0 International License, which permits any non-commercial use, sharing, distribution and reproduction in any medium or format, as long as you give appropriate credit to the original author(s) and the source, provide a link to the Creative Commons licence, and indicate if you modified the licensed material. You do not have permission under this licence to share adapted material derived from this article or parts of it. The images or other third party material in this article are included in the article’s Creative Commons licence, unless indicated otherwise in a credit line to the material. If material is not included in the article’s Creative Commons licence and your intended use is not permitted by statutory regulation or exceeds the permitted use, you will need to obtain permission directly from the copyright holder. To view a copy of this licence, visit http://creativecommons.org/licenses/by-nc-nd/4.0/ .
Reprints and permissions
About this article
Cite this article.
Kasoju, N., Sunilkumar, A. Convergence of tissue engineering and sustainable development goals. Biotechnol Sustain Mater 1 , 20 (2024). https://doi.org/10.1186/s44316-024-00021-y
Download citation
Received : 09 August 2024
Accepted : 27 November 2024
Published : 19 December 2024
DOI : https://doi.org/10.1186/s44316-024-00021-y
Share this article
Anyone you share the following link with will be able to read this content:
Sorry, a shareable link is not currently available for this article.
Provided by the Springer Nature SharedIt content-sharing initiative
- Bioengineering
- Biofabrication
- Regenerative medicine
- Medical devices
- Sustainability
Biotechnology for Sustainable Materials
ISSN: 2948-2348
- General enquiries: [email protected]
Thank you for visiting nature.com. You are using a browser version with limited support for CSS. To obtain the best experience, we recommend you use a more up to date browser (or turn off compatibility mode in Internet Explorer). In the meantime, to ensure continued support, we are displaying the site without styles and JavaScript.
- View all journals
Tissue engineering articles from across Nature Portfolio
Tissue engineering is a set of methods that can replace or repair damaged or diseased tissues with natural, synthetic, or semisynthetic tissue mimics. These mimics can either be fully functional or will grow into the required functionality.
Latest Research and Reviews
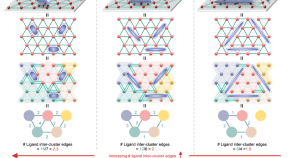
Modularity-based mathematical modeling of ligand inter-nanocluster connectivity for unraveling reversible stem cell regulation
The extracellular matrix is remodeled to form complex interconnected network structures that regulate stem cell behaviors. Here, the authors modulate extracellular matrix-like networks using magnetic nano-blockers, adjusting stem cell behavior by changing ligand inter-cluster connectivity. This dynamic system provides new insights into cell-material interactions for tissue regeneration.
- Nayeon Kang
- Heemin Kang
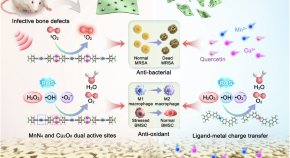
Biodegradable Janus sonozyme with continuous reactive oxygen species regulation for treating infected critical-sized bone defects
Existing biodegradable materials lack long-term therapeutical effect because of their gradual degradation. Here, the authors develop a degradable material with continuous ROS modulation, which rapidly eliminates bacteria and promotes bone regeneration in infected cranial defects.
- Xiaobo Feng
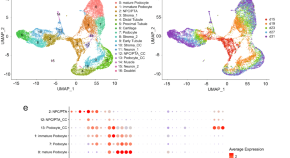
Proximal tubule cell maturation rate and function are controlled by PPARα signaling in kidney organoids
Single-cell RNA sequencing of Kidney organoids revealed varying maturation rates in proximal tubule cells, with PPARα signaling linked to faster maturation. PPARα signaling agonists promoted maturation and increased nephrotoxic drug sensitivity.
- Yoshiki Sahara
- Minoru Takasato
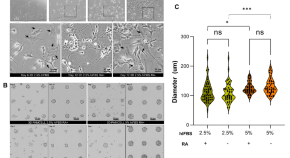
Development of a Disease Modeling Framework for Glutamatergic Neurons Derived from Neuroblastoma Cells in 3D Microarrays
- Duc Long Nguyen
- My Phuong Thi Le
- Huyen T. M. Pham
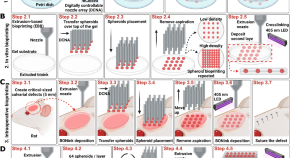
High-throughput bioprinting of spheroids for scalable tissue fabrication
HITS-Bio, a high-throughput bioprinting platform, rapidly assembles spheroids to mimic native tissue architecture. Its intraoperative application in bone shows near-complete calvarial defect repair in rats and fabrication of scalable cartilage.
- Myoung Hwan Kim
- Yogendra Pratap Singh
- Ibrahim T. Ozbolat

Unveiling the impact of hypodermis on gene expression for advancing bioprinted full-thickness 3D skin models
We used 3D bioprinting to create a full-thickness human skin model with a hypodermis layer, offering an in vitro tool for disease and toxicology studies. This model replicates skin function and highlights the hypodermis’ role in tissue development.
- Thayná M. Avelino
- Samarah V. Harb
- Ana Carolina M. Figueira
News and Comment
Developing sex-accurate cell culture environments.
The extracellular matrix remains under-recognized as a sex-dependent entity. Designing culture environments that account for sex as a biological variable requires considering not only cellular sex, but also the usage of sex-specific scaffolds to create a holistically sex-accurate platform.
- Alexandra N. Borelli
- Kristyn S. Masters
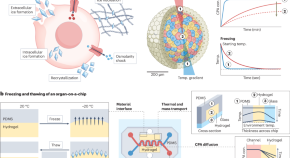
Cryopreservation of in vitro human tissue models
The widespread use and translation of engineered in vitro tissues, such as organoids, organ-on-chip devices and microphysiological models, will require designs that can be preserved by cryopreservation. Here, we outline the factors that affect their cryopreservation, highlighting the challenges that remain to be addressed.
- Yan Yan Shery Huang
Human intestinal immuno-organoids
- Chiara Anania

Towards a competitive cost for industrial-scale cultivated chicken production
A techno-economic analysis demonstrates that cultivated chicken can be produced for under US$7 per pound when bioprocesses are scaled to reach the required throughput. By using lab-scale experimental data and empirical correlations, this study unveils important factors that can render industrial-scale cultivated chicken production commercially viable.
- Dimitrios I. Gerogiorgis
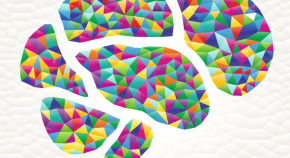
Chimeric brain organoids
An article in Nature reports the generation of human chimeric brain organoids that integrate cells from multiple donors.
- Caroline Beyer
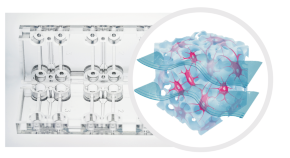
3D cell networks advance bone-on-a-chip
An article in Nature Communications presents a synthetic biodegradable void-forming hydrogel that supports in vitro formation of 3D networks from human primary cells for bone-on-a-chip applications.
Quick links
- Explore articles by subject
- Guide to authors
- Editorial policies


IMAGES
COMMENTS
Apr 29, 2022 · Tissue engineering is the process of producing artificial tissues. It is necessary for repairing tissues or replacing organs in the human body. This innovation has brought success in medicine since it has helped in the engineering of necessary tissues such as the skin, bone marrow, bones, and pancreas.
Tissue engineering is generating a new field of study in which the principles of engineering and biology to correct the damaged tissue, uses and can renewal, operation and maintenance of tissue healing. In order to use an ideal scaffold Tissue engineering should have features such as non-toxic Cell and tissue properties to be fit. About, bone ...
Tissue engineering is generating a new field of study in which the principles of engineering and biology to correct the damaged tissue, uses and can renewal, operation and maintenance of tissue healing. In order to use an ideal scaffold Tissue engineering should have features such as non-toxic Cell and tissue properties to be fit.
Tissue engineering is an emerging interdisciplinary field that uses principles from engineering, biology and chemistry in an effort towards tissue regeneration. The main draw of tissue engineering is the regeneration of a patient’s own tissues and organs free from low biofunctionality and poor biocompatibility and serious immune rejection.
Today tissue engineering is a thriving areas. As of 2011, there are over 100 companies involved in tissue engineering, employing 14,000 people and generating nearly $4 billion in sales [2]. Here we discuss some of the progress that’s been made and the challenges ahead. Liver and Vital Organs In our initial paper describing what is now known ...
This article will review the basic techniques used in tissue engineering and discuss some of the progress that has been achieved in this field. THE BASIC COMPONENTS OF REGENERATIVE MEDICINE STRATEGIES. The field of regenerative medicine encompasses various areas of technology, such as tissue engineering, stem cells, and cloning.
Tissue Engineering of Cardiovascular System. Ali Ostadfar PhD, in Biofluid Mechanics, 2016. 9.1 Introduction. Tissue engineering is the application of methods and principles of engineering, medicine and physiology to obtain novel tissues with similar functions and characters as a biological substitute for implantation into the body for the purpose of replacing, repairing or enhancing organ ...
4 days ago · Key aspects of tissue engineering. TE requires a triad of components: (1) Harvested and dissociated cells from the donor tissue; (2) scaffolds made of biomaterials on which cells are attached and cultured, then implanted at the desired site in functioning tissue; and (3) growth factors that promote and/or prevent cell adhesion, proliferation, migration, and differentiation by up-regulating or ...
Dec 3, 2024 · Tissue engineering is a set of methods that can replace or repair damaged or diseased tissues with natural, synthetic, or semisynthetic tissue mimics. These mimics can either be fully functional ...
Jan 1, 2020 · Traditional tissue engineering: Traditional tissue engineering includes two main approaches: (1) transplantation of a tissue grown in vitro consisting of an artificial matrix with cells and growth factors, and (2) in situ regeneration of tissue utilizing a combination of an artificial matrix and growth factors as a guiding template to induce host cell regeneration of the tissue in vivo (Fig. 2 ...